FIGURE 75-1. Graphic representation of the pathways of iodothyronine metabolism. The types 1 and 2 deiodinases (D1, D2) catalyze removal of the 5′ (or chemically equivalent 3′) iodine from T4 and other iodothyronine substrates. Types 1 and 3 enzymes catalyze 5 (or chemically equivalent 3) deiodination. A variety of less common reactions occur via alternative enzymatic pathways. The metabolites shown are subject to further deiodination to form diiodothyronines, monoiodothyronines, and tyrosine.
(From St. Germain DL, Galton VA: The deiodinase family of selenoproteins. Thyroid 7:655–658, 1997.)
The enzymatic processes shown in Fig. 75-1 are not mutually exclusive. Indeed, modification of an iodothyronine molecule at one site may markedly alter its susceptibility to other metabolic reactions. For example, Tetrac and Triac are much better substrates for glucuronidation than are T4 and T3.12 The acetic acid analogues and sulfated conjugates also are markedly better substrates for deiodination in the liver and kidney than are the native compounds.13,14 In contrast, sulfation of iodothyronines in certain organs may effectively block further metabolism because these conjugates are poorly reactive with the deiodinase isoforms expressed in other tissues.15,16
HORMONE KINETICS AND PRODUCTION
Deiodination at the 5′ or 5 position accounts for approximately 80% of the daily disposal of T4, with the other processes shown in Fig. 75-1 responsible for the remaining metabolism of this compound.5 Such approximations may underestimate the role of both deiodinative and nondeiodinative pathways, however. Many of the products of these reactions, including T3 and rT3, are present primarily in the intracellular compartment and may undergo degradation before they have a chance to exchange with the plasma pool. It thus is difficult in human kinetic studies that use plasma sampling techniques alone to assess the contribution of these processes to overall thyroid hormone metabolism. For example, it has been demonstrated that most of the thyronine (T0) excreted in the urine is in the form of its acetic acid analogue,17 thus suggesting that deamination plays a more prominent role in thyroid hormone metabolism than is apparent from the very low circulating levels of Tetrac and Triac. Such concerns have led to the concept that “hidden pools” of thyroid hormone metabolites may be present in tissues.18
Table 75-119–21 provides estimates of various kinetic parameters of thyroid hormone production and metabolism in humans.5,22,23 The high affinity of T4 for plasma binding proteins, along with its greater production rate, accounts for its relatively high concentration in serum, as well as its long serum half-life. In contrast, T3 and rT3 are present at much lower serum concentrations because of their lower production rates, greater metabolic clearance rates, and lower affinity for thyroxine-binding globulin (TBG). In addition, these two triiodothyronines appear to reside primarily within the intracellular compartment; thus their volumes of distribution are significantly greater than that of T4.
The rate of T4 production remains remarkably constant in healthy adults, with alterations noted only during pregnancy and in the aged. Although not well documented, increased T4 secretion probably occurs during pregnancy in response to several factors, including thyroidal stimulation by human chorionic gonadotropin, an increase in the size of the extrathyroidal T4 pool resulting from increased TBG levels, and an increase in the rate of T4 and T3 degradation because of high levels of 5-deiodinase activity in the pregnant uterus and placenta.24,25 One clinical consequence is the need to increase the replacement dose of T4 by 25% to 50% in hypothyroid women during pregnancy.26 Thyroid function appears to be well preserved through age 80 in healthy individuals, although a slight decrease in T4 production and clearance is noted after the age of 60 years, and free T3 levels are lower in centenarians.27,28 That being the case, the decreased T4 requirements of elderly hypothyroid patients probably result more from the presence of chronic illness and the use of concurrent medications than from the aging process per se.29,30
DEIODINATION AND THE IODOTHYRONINE DEIODINASES
The potential importance of deiodination to thyroid hormone action was first recognized nearly 50 years ago when Gross and Pitt-Rivers demonstrated that although T3 was considerably more potent than T4,31 it was present in the thyroid in much lower amounts.32 This observation suggested that T3 was derived largely from T4 by metabolism in extrathyroidal tissues. This thesis was later proved by Braverman et al., who demonstrated the presence of T3 in the serum of athyreotic subjects injected with T4.33 Research over the past three decades has confirmed the physiologic importance of the 5′- and 5-deiodination processes, has defined the biochemical parameters of these enzymatic reactions, has determined important regulatory factors that influence deiodinase activities, and has identified key structural determinants of the proteins that catalyze these reactions.34,35
Three deiodinase isoforms, termed D1, D2, and D3, are present in vertebrate species. These differ in their catalytic properties, patterns of tissue expression, and mechanisms of regulation.34,36,37 The 5′- and 5-deiodination reactions catalyzed by these enzymes can be considered broadly as activating and inactivating processes, respectively.
BIOCHEMICAL CHARACTERISTICS
The biochemical properties of the deiodinases are outlined in Table 75-2.38–45 They are in essence oxidoreductases in that they catalyze the substitution of hydrogen for iodine on the iodothyronine substrate. No other catalytic properties of these enzymes have yet been identified, nor do other known enzymes possess deiodinase activity. The enzymes are remarkable in terms of their substrate specificity and the precise location of the iodine removed.16 D1 is unique in that it can catalyze either 5′- or 5-deiodination, depending on the reacting substrate. Thus rT3 is efficiently deiodinated only at the 5′ position by D1. In contrast, T4 and T3 are poor substrates for this enzyme unless they are first sulfated. This reaction markedly enhances their rate of 5-deiodination and further reduces their susceptibility to 5′-deiodination.46 That D1 is relatively inefficient in converting T4 to T3 presents a considerable paradox, given that a significant proportion of T3 production has traditionally been believed to occur in the liver and other D1-expressing tissues (vide infra).
D2 catalyzes only 5′-deiodination and very efficiently converts T4 to T3.16 The T3 thus formed is a poor substrate for 5′-deiodination and is not further metabolized by this enzyme. In contrast, rT3 is a good substrate for D2 as well as D1 and frequently is used in research assays to quantitate 5′-deiodinase activity. D3 exclusively catalyzes 5-deiodination16 and thus serves to convert T4 and T3 to rT3 and 3,3′-T2, respectively—metabolites with little affinity for the nuclear thyroid hormone receptors.
The deiodinases all require the availability of reduced thiol cofactors for efficient catalytic cycling.39 These cofactors presumably function to displace iodine from an enzyme intermediate formed during the reaction and thus to regenerate the active deiodinase.47 In in vitro assay systems using tissue homogenates or cellular subfractions, dithiothreitol typically is added as a cofactor. This small, nonnative four-carbon dithiol efficiently supports deiodination of all three enzymes. Kinetic data derived from broken cell preparations using dithiothreitol as cofactor have demonstrated a Michaelis-Menten constant (Km) value for D1 of approximately 2.3 µmol/L (for T4 5′-deiodination), whereas D2 and D3 manifest much lower Km values in the nanomolar range (see Table 75-2).39 Based on this analysis, D1 sometimes is referred to as a “high Km” enzyme, whereas D2 is said to catalyze a “low Km” 5′-deiodination process. This distinction is somewhat spurious, however, because the kinetic properties of the deiodinases are clearly dependent on the thiol cofactors used in the assay system.48 Thus, when the native thiol cofactor glutathione or thioredoxin is used, Km values for D1 in the nanomolar range are obtained. Unfortunately, the cofactor system supporting deiodination in intact cells remains unknown,49 and evidence suggests that glutathione and thioredoxin may not serve this role.50 Thus the physiologic significance of the kinetic parameters derived in vitro remains uncertain.
INHIBITORS
In addition to differences in their catalytic properties, the deiodinases show differential susceptibilities to certain inhibitors39 (see Table 75-2). Most notable is the marked sensitivity of D1 to the antithyroid drug propylthiouracil (PTU). This agent forms an inactive complex with D1 by binding covalently to its active site. Notably, D2 and D3 show little or no susceptibility to inhibition by PTU. The related thioureylene drugs carbimazole and methimazole have no inhibitory effect on deiodination.47
Gold compounds such as aurothioglucose are known to react with the active site selenocysteine residue in glutathione peroxidase and impair its activity.51 This compound also inhibits all three deiodinase isoforms, with D1 again showing a significantly greater sensitivity to this effect.52–54 Other drugs that affect thyroid hormone metabolism include the iodinated radiographic contrast agents iopanoic acid (Telepaque) and sodium ipodate (Oragrafin). These small phenolic compounds act as substrate analogues and inhibit all three deiodinases in a competitive manner. To date, no selective inhibitors of D2 or D3 have been described—a situation that has significantly limited experimental investigations into their physiologic roles.
TISSUE PATTERNS OF EXPRESSION
An intriguing feature of the deiodinases is their pattern of tissue expression (see Table 75-2). The liver, kidney, thyroid gland, and pituitary gland express high levels of D1, with lesser amounts found in the brain.16,55,56 In contrast, D2 is most abundant in the pituitary gland and brown adipose tissue (in rodents), with significant amounts also noted in the central nervous system.16 Studies using in situ hybridization and immunocytochemistry have demonstrated that D2 expression in the brain appears to be confined to certain subpopulations of astroglial cells, such as the tanycytes lining the third ventricle of the hypothalamus, which suggests that they serve as the principal site of T3 production in this tissue.57–59 In humans, D2 expression appears more widespread; the mRNA for this enzyme has been noted in the heart, skeletal muscle, and thyroid gland,54,60 as well as in coronary artery smooth muscle cells.61
In adult mammals, D3 expression occurs primarily in the brain, with significant amounts also present in the skin.62,63 The brain is thus the only tissue that expresses all three deiodinases, although these are generally found in different subregions of this tissue and in different cell types. Thus, as opposed to the expression of D2 by glial cells, D3 is expressed primarily in neurons.64,65
Finally, as detailed below, deiodinase expression patterns during pregnancy and development are of critical importance, with high levels of D3 activity present in the uterus, the placenta, and several fetal tissues.66,67
STRUCTURAL CHARACTERISTICS
Important structural features of the deiodinases have been deduced from the results of molecular cloning experiments.37 All the enzymes have a molecular mass of approximately 29 to 32 kDa, function as homodimers,43–45 and are selenoproteins in that they contain the uncommon amino acid selenocysteine as the reactive residue in the catalytic cleft (Fig. 75-2).68 The importance of this amino acid to enzymatic activity is demonstrated by experiments in which selenocysteine has been replaced by cysteine. Such a substitution decreases the catalytic efficiency of the mutant protein to less than 1% of that of the native enzyme.69 In the case of D3, the cysteine mutant also demonstrates an altered substrate preference.70 It is remarkable that substitution of selenocysteine for a serine residue in a monoclonal antibody raised against T4 confers on the protein deiodinase activity with catalytic properties similar to the D1, including sensitivity to PTU inhibition.71
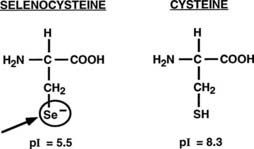
FIGURE 75-2. Comparison of the structures of selenocysteine and cysteine. Selenocysteine is ionized at physiologic pH, which likely contributes to the catalytic efficiencies of the deiodinases.
The importance of selenocysteine to deiodination likely derives from its being ionized at physiologic pH, thus serving as a much more potent nucleophile than cysteine.72 Incorporation of selenocysteine into the deiodinases and other selenoproteins occurs at the time of translation and is directed by a specific stem-loop structure in the 3′-untranslated region of the mRNA termed a selenocysteine insertion sequence.73 A unique tRNA (Sec-tRNA[Sec]), a specific RNA binding protein, and a specialized elongation factor also are required for efficient synthesis of selenoproteins.74,75
In earlier studies, it was suggested that a 29 kDa (p29) nonselenoprotein, almost identical in sequence to a member of the Dickkopf protein family (Dkk3), was a substrate binding subunit of the D2.76 However, Montero-Pedrazuela et al. have demonstrated a marked difference in spatial expression patterns in the brain of the p29 and the D2 selenodeiodinase,77 and as recently reported, mice deficient in the Dkk3 protein do not have any consistent alterations in thyroid hormone status or D2 activity in their tissues.78 These findings strongly suggest that the p29 protein is not relevant to D2 activity or to thyroid hormone homeostasis.
The overall amino acid identity of the three deiodinase isoforms is less than 30%. However, a high degree of homology is present in the regions of the selenocysteine and a conserved histidine residue (Fig. 75-3A). These areas show significant structural similarities to the thioredoxin-fold family of proteins and to α-l-iduronidase, respectively.79 Based on these structural homologies, Callebaut et al.79 and others have reported mutagenesis studies that define further the structure-function correlates of the deiodinase isoforms, including the identification of a key serine residue in the D1 active catalytic site that appears to confer sensitivity to PTU.80
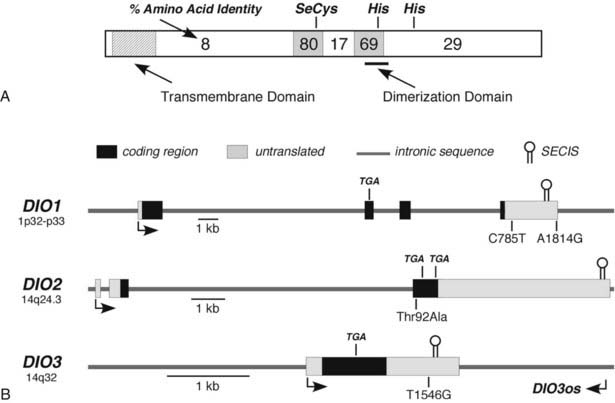
FIGURE 75-3. Structural features of the deiodinase proteins and genes. A, The proteins are composed of 257 to 278 amino acids with predicted molecular masses of 29 to 32 kDa. The hydrophobic transmembrane domain near the amino terminus, the selenocysteine residue (SeC), and two histidines (His) essential for catalytic activity are conserved in all three isoforms. The percentage of amino acid identity between the three isoforms in different portions of the molecule is given. A high degree of homology is noted in the regions surrounding the selenocysteine and one of the histidines. The dimerization domain as defined in the D1 is also shown. B, The structures of the genes, including the locations of the selenocysteine-encoding TGA codons and the regions coding for the selenocysteine insertion sequences (SECIS) located in the 3′-untranslated regions, are shown. Polymorphisms identified in each of the genes are also shown. DIO3os refers to the gene that encodes transcripts from the opposite DNA strand in the DIO3 locus and that remains incompletely characterized.
In addition, all deiodinases contain a hydrophobic region near the N-terminus,81 and subcellular fractionation studies have confirmed that these enzymes are integral membrane proteins.82,83 Such studies, along with fluorescent microscopy of epitope-tagged enzymes, have demonstrated that the D2 is located in the endoplasmic reticulum,82,84 whereas a significant fraction of the D3 has been reported to be in the plasma membrane.85 The location of the D1 may vary in different tissues; in renal epithelial cells, the D1 has been localized to the basolateral plasma membranes,86 whereas in the liver, it appears to reside in the endoplasmic reticulum (Pallud, Croteau, and St. Germain, unpublished observations). Studies by Friesema et al.87 demonstrate that the catalytic effectiveness of all three deiodinases is markedly increased when specific thyroid hormone transporters are coexpressed with the deiodinases in cultured cells. This finding indicates that the active catalytic sites of these enzymes are located in the intracellular compartment.
GENETIC CONSIDERATIONS AND KNOCKOUT MOUSE MODELS
The three deiodinase isoforms are coded in mammals by three different genes located in humans on chromosomes 1 (D1) and 14 (D2 and D3), as indicated in Table 75-2.40–42 Although their genetic structure appears relatively simple in that they contain only four, three, and a single exon, respectively (Fig. 75-3B),40,88,89 surprising complexities that may influence expression patterns have been identified.90,91 For example, the DIO3 gene locus also expresses antisense transcripts from the DIO3os gene coded on the opposite DNA strand, and in the mouse fetus, the D3 is imprinted; transcription preferentially occurs from the paternal allele.91 In humans the DIO3 is also located within an imprinted region of chromosome 14.91 This pattern of organization and expression of the D3 often is observed for genes important in development.92
To enhance our understanding of the physiologic roles of the deiodinases, genetic knockout models of each of the isoforms have been developed and partially characterized (Table 75-3).93–100 D1-deficient mice show no general phenotypic abnormalities, although serum T4 and rT3 levels are modestly elevated, and the excretion patterns of iodothyronines in the feces and of iodine in the urine are significantly altered.93 D2-deficient mice are viable and fertile in a protected laboratory setting. However, observed impairments in hearing,96 thermogenesis,95 and neurocognition97 in these mutants would likely result in lethality in the wild. These animals also manifest elevated T4 and thyroid-stimulating hormone (TSH) levels and show a marked selective resistance to the feedback effects of T4, demonstrating the importance of D2 in pituitary thyrotroph regulation.94 This contrasts with D1-deficient mice, in which TSH levels are normal.93 This implies that the D1 expressed in the pituitary is not involved in TSH regulation.
Table 75-3. Phenotypic Features of Deiodinase Knockout Mice
D1-Deficient Mouse93 |
D2-Deficient Mouse |
D3-Deficient Mouse99,100 |
A striking finding in the D1- and D2-deficent mouse models is that serum T3 levels are normal.93,94 Indeed, in mice cross-bred to yield a combined D1 and D2 deficiency, the serum T3 level is only minimally decreased (V. A. Galton, unpublished observations).101 This finding is both unexpected and difficult to explain, given that 5′-deiodination in peripheral tissues is supposed to contribute 80% of the T3 production in humans and 45% in rats, with the remainder secreted directly from the thyroid.34,102 Given that the combined D1/D2 knockout mouse has been unequivocally shown to lack all 5′-deiodinating capacity, it is clear that at least in the rodent, thyroidal production and secretion of T3, perhaps combined with a decreased rate of T3 clearance from D1 deficiency, can compensate and maintain serum T3 levels that are essentially normal in the face of an inability to convert T4 to T3 in all tissues. A surprising corollary to this observation is that a D1 deficiency does not appear to significantly compromise T3 secretion from the rodent thyroid, a tissue in which this enzyme is highly expressed and previously was thought to be essential for T3 production.102,103 This suggests that under physiologic conditions, D1 acts primarily as a “scavenger” enzyme that prevents the buildup of lesser iodothyronines (rT3, T2s, T1) and sulfated derivatives in the thyroid gland, blood, and tissues.
The extent to which humans may be able to compensate for at least a partial impairment in 5′-deiodinating capacity has recently been reported. Although to date no mutations in the deiodinase genes in humans have been described, Dumitrescu et al.104 have described two families with partially inactivating mutations in the selenocysteine insertion sequence-binding protein2 (SBP2), which is essential for the synthesis of selenoproteins, including the deiodinases.74 Affected individuals demonstrate short stature and delayed bone age along with modest abnormalities in serum thyroid hormone levels, including increases in total T4 and rT3.104 Notably, total serum T3 levels were only minimally reduced, whereas TSH levels were at the upper part of the reference range and proved relatively resistant to suppression by administered T4, but not T3. These findings are very similar to those observed in knockout mice with a combined deficiency in D1 and D2, as noted previously. Indeed, a deficiency in D2 activity was demonstrated in cultured skin fibroblasts from affected patients.104
Mice deficient in D3 present the most severe phenotype, likely as a result of the importance of this enzyme during the developmental period. Observed abnormalities include significant perinatal mortality, growth retardation, impaired fertility, and altered systemic thyroid hormone levels during the perinatal period (transient hyperthyroidism) and in adulthood (moderate hypothyroidism).99,100 Multiple abnormalities, including impaired response of the thyroid gland to TSH and of the pituitary to TRH, have been observed at all levels of the thyroid axis in the D3-deficient adult mouse. The response to hypothyroidism of TRH mRNA production in the hypothalamus and of pituitary TSH production is also markedly impaired. Many of these abnormalities are similar to those observed in human infants born to mothers with poorly controlled hyperthyroidism during pregnancy105,106 or to rodents treated with large doses of thyroid hormone during the perinatal period.107,108 These findings demonstrate that strict control of thyroid hormone levels during development by D3 is critical for proper development of the thyroid axis.
REGULATION
The deiodinases are regulated by multiple hormones, growth factors, and environmental and nutritional factors.34 Foremost among these are the thyroid hormones themselves; alterations in thyroid status induce profound changes in enzyme activity (see Table 75-2). Hypothyroidism is associated with a marked decrease in D1 and D3 levels, whereas D2 activity increases severalfold. Opposite changes occur in hyperthyroidism. These changes result from both pretranslational and posttranslational mechanisms.34 For example, D1 and D3 mRNA levels are increased in the hyperthyroid state,53,109 and D2 mRNA is decreased.54 Hyperthyroidism also results in rapid downregulation of D2 activity by ubiquitination of the D2 protein.110,111 This induces a reversible conformational change in the enzyme’s dimerization structure, which results in reversible loss of activity and eventually may target the enzyme for proteasomal degradation.112 Considerable progress has been made in defining the molecular mechanisms involved in transcriptional control of the deiodinases; this topic has been reviewed recently by Gereben et al.37 Of particular interest has been the observation by Simonides et al.113 that hypoxia induces D3 expression in several cell culture systems, as well as in ischemic myocardium.
Other important regulatory effects on deiodinase activity are noted in the thyroid gland, where TSH and thyroid-stimulating immunoglobulins stimulate both D1 and D2 activity60; in brown adipose tissue, where cold exposure,114 bile acids,115 or intracerebroventricular administration of leptin116 markedly stimulates D2 activity; and in the liver, where nutritional deprivation, diabetes, tumor necrosis factor, and other cytokines decrease D1 activity (see also below).109,117,118 In addition, D2 activity in the brain displays a significant diurnal variation.119 Although the physiologic significance of this remains to be determined, in some seasonal breeding species, rhythmic alterations in D2 and D3 activity in the central nervous system, perhaps mediated by melatonin,120 appear to be integral responses to changes in the photoperiod.121,122
Alternative Routes of Iodothyronine Metabolism
In addition to deiodination, iodothyronines are metabolized by multiple other mechanisms.123 In this regard, conjugation of the phenolic ring hydroxyl group to sulfate or glucuronide probably represents the second most prevalent mechanism of thyroid hormone metabolism.16 Sulfation and glucuronidation are inactivating reactions because the compounds formed are devoid of thyromimetic activity, do not bind to nuclear thyroid hormone receptors, and are rapidly metabolized by D1 or excreted in bile.4,16 However, sulfation is a reversible process that occurs through the action of tissue sulfatases or bacterial sulfatases in the intestine.124 Thus T3 sulfate (T3S) injected into hypothyroid rats demonstrates approximately 20% of the thyromimetic activity of native T3 because of liberation of this active hormone.125
Sulfotransferases in the cytoplasm of many tissues serve to catalyze the formation of iodothyronine sulfate conjugates. In rat tissue homogenates, 3,3′-T2 and T3 appear to be the best substrates for sulfation.46 Although the exact enzymes mediating iodothyronine sulfation are uncertain, the phenol sulfotransferases found in human liver and kidney have been demonstrated in vitro to possess this activity.126 Sensitive and specific radioimmunoassays for various iodothyronine sulfates have been developed and used to demonstrate detectable but very low levels of T3S, rT3S, and T4S in normal adult human serum.46 These low circulating levels probably reflect the rapid metabolism of these compounds by D1. Evidence for this thesis comes from experiments in animals and humans, in which treatment with inhibitors of D1 activity, such as PTU or iopanoic acid, results in marked increases in iodothyronine sulfoconjugate levels in both serum and bile.127,128 In other circumstances where D1 activity is low or impaired, such as during fetal life, in hypothyroidism, or in nonthyroidal illness, serum levels of these compounds also are elevated, either in absolute terms or relative to the native, unconjugated iodothyronines.16 To date, the physiologic role of sulfation in thyroid hormone economy has not been clearly defined, although it appears to represent an important component of the degradative process.
Glucuronidation of iodothyronines followed by their secretion into bile represents another pathway of iodothyronine clearance. Studies in humans, however, suggest that this pathway accounts for less than 1% of the total clearance of T4 by the liver, and only minute amounts of these conjugates are present in plasma.5,129
Two enzymes, l-amino acid oxidase and thyroid hormone aminotransferase, have been implicated in catalyzing the deamination of T4 and T3 to their acetic acid analogues Tetrac and Triac, respectively.130 Current evidence suggests, however, that these reactions occur to only a limited extent in normal humans. Triac has intrinsic biological activity equivalent to that of T3 when tested in in vitro assay systems,130 and it binds with greater avidity than T3 to the β isoform of the thyroid hormone receptor.1 Injection of Triac into humans or animals results in significant physiologic effects; however, relatively large doses are required because of its apparent rapid clearance and degradation via deiodination and glucuronidation.131 Despite its short half-life, Triac has been used successfully in the treatment of thyroid hormone resistance states.1 As in the case of sulfated conjugates, levels of Triac and its conjugates increase in circumstances in which D1 activity is low or impaired, such as during fasting or treatment with PTU or iopanoic acid.130 The physiologic relevance of these observations is uncertain but could be important, given Triac’s significant intrinsic activity. As noted earlier, the novel compound 3-iodothyronamine, formed by sequential deiodination and decarboxylation of thyroid hormones, may have important physiologic effects in some species, although its role in humans has not yet been defined.10,11
A final mechanism of thyroid hormone metabolism involves oxidative cleavage of the ether link of T4 and T3 by phagocytosing leukocytes.132 Current evidence suggests that this pathway is a minor one for iodothyronine degradation, except in the special circumstances of severe bacterial infection.
Thyroid Hormone Uptake Into Cells
Although some nongenomic effects of thyroid hormones may be triggered directly by hormones residing within the extracellular space via binding to cell surface receptors,133 the effects of these compounds on gene transcription, as well as on other cellular events, require their uptake into the cellular compartment. Similarly, the metabolic processes described above likely take place in various intracellular locations.87 Thus, the uptake of thyroid hormones into cells is a prerequisite for their affecting physiologic functions and for their metabolism.
Several membrane proteins capable of transporting thyroid hormones into and perhaps out of cells have been identified recently.134 Members of the organic anion-transporting polypeptide (OATP) family135 and the monocarboxylate transporter (MCT) family136 have been best characterized with regard to their capacity to affect cellular uptake and facilitate metabolism of various thyroid hormones. OATP1C1 has a relatively high affinity for T4 and rT3 and is highly expressed in capillaries in the rodent brain, suggesting a role in the transport of T4 across the blood-brain barrier. It also is widely expressed in the human brain.137 The MCT8 transporter facilitates cellular uptake of T3 and T4 when expressed in Xenopus laevis oocytes and cultured COS1 cells, and results in marked enhancement in the rate of iodothyronine metabolism by the deiodinases.87,138 The transporter is expressed in the choroid plexus and neuronal cells in the brain, as well as in the liver, kidney, heart, and placenta.134 It also appears to facilitate thyroid hormone exit from cells, which is likely important for overall cellular homeostasis.134
Recently, knockout mouse models of MCT8 deficiency have been described that verify the importance of this transporter in thyroid hormone homeostasis.139,140 Key features of these mice include elevations in serum T3 level, decreases in serum T4 and rT3 levels, and decreased brain content of T3. Deiodinase activities are also altered in several tissues secondary to tissue-specific patterns of thyroid hormone uptake. Thus, D1 expression is increased in the liver as the result of thyrotoxicosis in that organ (despite MCT8 deficiency) from high serum T3 levels, whereas the relative brain hypothyroidism results in elevated D2 activity and decreased D3 activity in that tissue. It is surprising to note that the neurologic phenotype of MCT8-deficient mice appears to be mild. This contrasts sharply with the severe phenotype of humans bearing mutations in MCT8, which results in the Allan-Herndon-Dudley syndrome.141 Male patients with this disorder (the MCT8 gene is located on the X chromosome) display poor muscle tone, an inability to speak, and severe mental retardation. Serum thyroid hormone parameters in these patients are analogous to those observed in MCT8-deficient mice. The striking differences in neurological phenotype between the two species is as yet unexplained.
An Integrated View of Thyroid Hormone Metabolism
The preceding discussion has served to highlight the extraordinary complexity of thyroid hormone metabolism. Two critical features of this intricate system are apparent. First, the uptake and metabolic fate of iodothyronines vary significantly in different organs and even in different cell types within an organ, thus providing multiple mechanisms whereby thyroid hormone content and action can regulate differential metabolic control over physiologic processes. Second, these metabolic processes and in particular the rates of deiodination are regulated by several factors, which suggests that alterations in thyroid hormone metabolism may be important in adapting to internal and external homeostatic challenges.
ALTERED THYROID STATES
Several examples of these principles can be cited, starting first with the response to alterations in thyroid hormone status. Experimental studies, as well as clinical experience, indicate that serum T3 levels tend to be maintained within the normal range in patients with moderate degrees of hypothyroidism despite the attendant hypothyroxinemia.142,143 Several factors, including an increase in the relative proportion of T3 secreted from the thyroid gland and an increase in the proportion of T4 converted to T3 in extrathyroidal tissue, appear to be responsible for this finding144 (Fig. 75-4). Both of these effects result from increased rates of 5′-deiodination. Thus in the thyroid gland, D1 and D2 activities are stimulated by the increase in TSH that accompanies hypothyroidism.60 In peripheral tissues, T4 to T3 conversion mediated by D2, which recently has been postulated to be the major source of plasma T3 in euthyroid humans,145 is also relatively enhanced by increased D2 activity.54 As a result, T4 is used more efficiently for T3 production. In addition, the rate of T3 clearance in extrathyroidal tissues is decreased. This decrease probably results in part from diminished 5-deiodination caused by the decreases in D1 and D3 activity that have been observed in the hypothyroid state. The physiologic importance of these extrathyroidal mechanisms, which in essence act as a form of peripheral autoregulation to help maintain T3 levels, is easily demonstrated by the administration of varying doses of T4 to athyreotic individuals. Under such circumstances, the circulating T3/T4 ratio is highest when low doses of T4 are given, and the ratio progressively declines as full replacement and then supraphysiologic doses are provided.142,143 Alterations in the rates of nondeiodinative pathways may contribute to this response. The net effect of these metabolic adaptations is to minimize the decrease in circulating T3 levels in the face of impaired secretion from the thyroid gland.
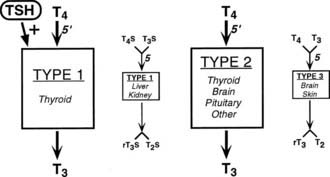
FIGURE 75-4. Autoregulation of thyroid hormone metabolism that accompanies the hypothyroid state, as might occur in iodine deficiency. D1 activity in the thyroid is stimulated by thyroid-stimulating hormone (TSH). Although this enzyme is relatively inefficient in converting T4 to T3, in the high T4 environment of the thyroid gland, this may serve to increase the proportion of T3 formed and secreted by this organ. Concurrently, D1 activity in extrathyroidal tissues is reduced, thereby decreasing the degradation of T4 and T3 by the 5-deiodination of their sulfated analogues. D2 activity is increased in all tissues that express this enzyme, which increases the proportion of T4 to T3 conversion in both the thyroid and peripheral tissues. Finally, D3 activity is decreased, again serving to diminish T4 and T3 degradation by 5-deiodinase processes. The net effect of these changes is to increase the relative rate of T3 production and decrease T3 degradation, thereby preserving the circulating and tissue levels of this active hormone in the presence of a diminished supply of T4.
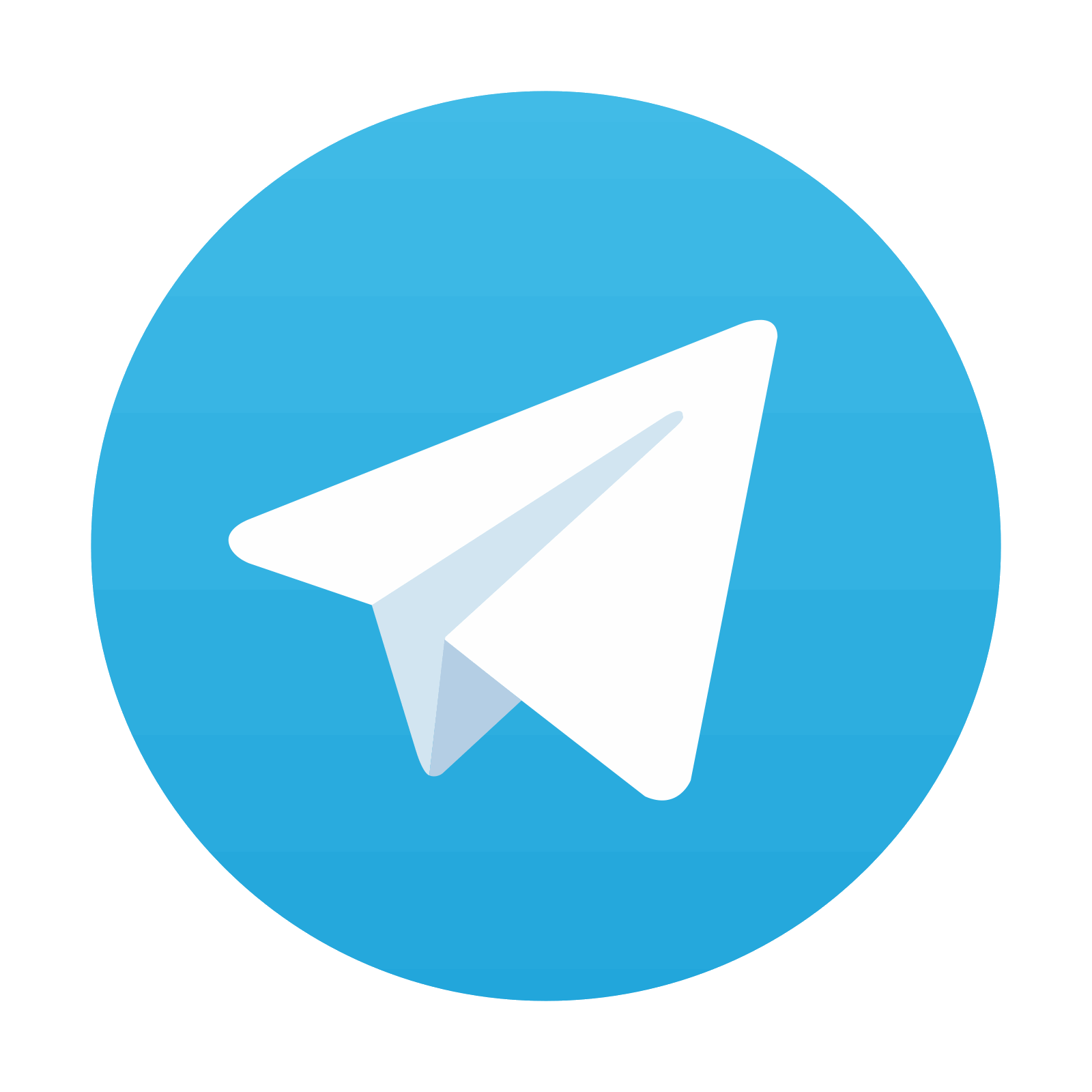
Stay updated, free articles. Join our Telegram channel
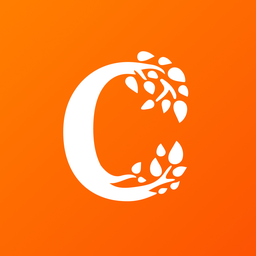
Full access? Get Clinical Tree
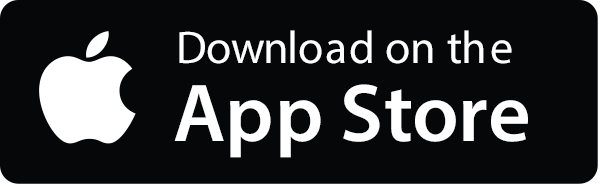
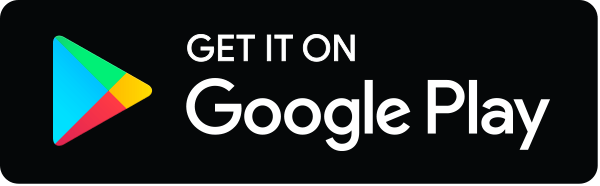