FIGURE 136-1. Comparison of testicular components and cells from Asian (A), Hispanic (B), and white (C) men.
Based on data from ref. 5.
Spermatogenesis
Mammalian spermatogenesis, including in humans, is a complex, dynamic process that depends on the proliferation and maturation of germ cells derived from self-renewing stem cells. Throughout life, the stem cells maintain a supply of germ cells capable of differentiating into fully formed spermatozoa. The duration of the entire process of spermatogenesis varies between species6—for example, 35 days in the mouse and hamster, 50 days in the rat, 45 to 65 days in various nonhuman primates, and 70 days in humans.6 In humans, the onset of release of spermatozoa (spermarche) occurs in the early stages of puberty at approximately 13.5 years of age,7 and spermatogenesis continues throughout life.8 The quantitative output of spermatozoa in an adult man is remarkable and equivalent to approximately 1000 new sperm per second, with 150 to 250 × 106 sperm commonly present in a single ejaculate. The notion of the testis as a biological factory is based upon its similarity to a production line of many different components, modified and adjusted for ultimate assembly into its finished product, namely the spermatozoa. Although the rate of sperm production in the adult human is impressive, this level of activity is not unique to the testis. Similar, if not even greater, cell production is shown by the bone marrow and the epithelium of the gastrointestinal tract. Like the seminiferous epithelium, these tissues produce terminally differentiated cells that are lost and therefore must be continuously replaced throughout a lifetime. Injury, disease, or mutations all may negatively impact spermatogenesis, but our current understanding of the mechanisms that promote or impair sperm production is limited, owed in part to the challenge of unraveling the complex and dynamic organization of somatic and germ cells and multiple interactions between them.
SEMINIFEROUS TUBULES
In man, the seminiferous tubules are approximately 200 µm in diameter with a total length of about 600 meters, occupying approximately 60% of testis volume. Structural support of tubules is provided by peritubular tissue consisting of five to seven layers of myofibroblasts and fibroblasts separated by collagen and extracellular matrix.9 Transport of sperm along the seminiferous tubules toward the rete testis occurs via peristaltic contraction/relaxation of peritubular tissue, a process regulated by the local production and action of vasoconstrictive and vasorelaxant agents such as endothelin, vasopressin, oxytocin, and nitric oxide.10–12 Peritubular cells are androgen responsive and secrete various proteins capable of modifying the intratubular environment and maintaining interactions between the basement membrane and the seminiferous epithelium.13,14
SEMINIFEROUS EPITHELIUM
Germ cells occupy most of the seminiferous epithelium, and their basal-to-luminal arrangement in multiple layers is supported structurally by the tall columnar Sertoli cells. The histologic relationship between the nondividing Sertoli cells and the various types of dividing and differentiating germ cells is difficult to appreciate by light microscopy, since the cytoplasmic extensions of Sertoli cells between the germ cells are thin. The complexities of this association have been thoroughly described in ultrastructural studies (see reviews elsewhere15,16). As germ cells progress through spermatogenesis, they are progressively transported apically through the seminiferous epithelium within pockets or recesses of Sertoli-cell cytoplasm and ultimately released, as spermatozoa, into the lumen of the seminiferous tubule (Figs. 136-2 to 136-4). Although the histologic appearance of the seminiferous epithelium is fundamentally similar in all mammals, including primates and humans, species variations do occur in the relationships between particular groups or associations of germ cells and the Sertoli cells within defined segments of the seminiferous tubules. These characteristic associations are discussed in the section on the spermatogenic cycle. The complexity of the spermatogenic process is evident from the following considerations: (1) dependence of the seminiferous epithelium on appropriate stimulation of the testis by gonadotropins, steroid hormones (notably androgens), and local paracrine, autocrine, and growth factors; (2) differential expression of genes within germ cells and Sertoli cells; (3) interactions between individual Sertoli cells and the various types of developing germ cells with which they are intimately associated; and (4) maintenance by the Sertoli cells of a unique physiologic environment within the seminiferous tubule that restricts the entry of cells, macromolecules, and immunogenic agents originating from the intertubular tissues and vasculature. Disturbance or impairment of any one of these parameters may result in disruption or suppression of spermatogenesis and contribute to defects in spermatozoa and/or a decrease in sperm count (see Chapter 142).

FIGURE 136-2. Drawing of the human seminiferous epithelium shows the relationship between the Sertoli cells and the position of germ cells. Type A pale (Ap), A dark (Ad), and B spermatogonia (B) are shown, together with primary spermatocytes (P), early round spermatids (RS), and elongating spermatids (ES). Inter–Sertoli cell tight junctions are indicated by arrows.

FIGURE 136-3. Seminiferous epithelium of the rat testis illustrates Sertoli cell nuclei (S), spermatogonia (Sg), primary spermatocytes (Sc), step 8 spermatids (8), step 19 spermatids (19), and peritubular tissue (PT).

FIGURE 136-4. Sequence of germ cell types in human spermatogenesis commencing with a spermatogonium, progressing through an orderly sequence of cell proliferation and maturation, and terminating in a mature spermatozoon. Spermatogonia: types A dark (Ad), and B (B); primary spermatocytes: leptotene (L) and pachytene (P); spermatids (Sa, Sc); peritubular tissue (PT).
SPERMATOGONIA AS STEM CELLS
The continuous production of spermatozoa that are released from the seminiferous epithelium requires that successive new generations of germ cells be made available to replenish those that leave the testis. Spermatogenesis in mammals commences with the replication of spermatogonia, which are associated with the basement membrane of the seminiferous epithelium. Most of our knowledge concerning the identity, relative numbers, and proliferative and maturation capabilities of spermatogonia, together with the factors that control their activities, is based on studies of laboratory species.17–19 In all mammals studied to date, including primates20,21 and humans,22 spermatogonia are subdivided into types A and B according to nuclear chromatin patterns in histologic preparations. In mice and rats, intermediate-type spermatogonia are present, with morphologic features between those of types A and B.
The identification and characterization of the stem-cell population within the spermatogonial compartment has been clarified by recent data. These data aimed at (1) characterization of the true self-renewing germline stem cells that underpin fertility, (2) possible purification, expansion, and preservation of stem-cell lines available for use in the management of infertility, (3) an understanding of the roles of these cells in the development of testicular cancer, and (4) their relationship to embryonic stem cells and induced pluripotent stem cells, raising the possibilities that these cells may be capable of reprogramming for therapeutic purposes.
The morphology and activity of undifferentiated spermatogonia (i.e., stem cells) in the adult mouse testis has been extensively studied23; using morphologic criteria, they are designated as Asingle (isolated cells; As), Apaired (interconnected pairs; Apr), and Aaligned (chains of 4 to 16 or 32 spermatogonia; Aal). Type Asingle have long been considered the actual stem cells that self-renew in the stem-cell niche.24 With the application of new pulse-labeling methods using tamoxifen-inducible Cre recombinase coupled to lacZ expression from a reporter gene, a subset of undifferentiated Asingle spermatogonia has been reported. These cells retain the potential to self-renew but normally do not do so; instead they are transit-amplifying cells with rapid turnover.25 It is suggested that these potential spermatogonial stem cells are capable of restoring spermatogenesis in the event that the actual stem-cell niche is compromised. They express neurogenin 3 (Ngn3), a transcription factor26 (Fig. 136-5).

FIGURE 136-5. A model of the spermatogenic stem-cell compartments. (A) In the normal, steady-state spermatogenesis, the stem-cell potential is not limited to the cells that actually self-renew in the stem-cell niche (actual stem cells); some of the transit-amplifying cells that do not self-renew may also possess the potential (potential stem cells). Both of these compartments are included in the undifferentiated spermatogonia entity. (B) Upon loss of the actual stem cells, the potential stem cells would switch their mode from transit amplification to self-renewal, resulting in the genesis of the new actual stem cells (upward arrow). The emptied stem-cell niche may play crucial roles in triggering this shift of mode.
(From Yoshida S, Nabeshima Y, Nakagawa T: Stem cell heterogeneity. Actual and potential stem cell compartments in mouse spermatogenesis, Ann N Y Acad Sci 1120:47–58, 2007.)
The initiation of spermatogenesis seems not to rely upon the class of Asingle self-renewing spermatogonial stem cells thought to be the source of all other spermatogonia in the adult testis.27 In the mouse, the first round of spermatogenesis is established directly from gonocytes (Ngn3 negative) that in turn give rise to differentiating spermatogonia, thus bypassing the requirement for Asingle stem cells.27 Subsequent rounds of spermatogenesis are derived from Asingle stem cells.
Spermatogonial Stem-Cell Niche
The concept of a niche denoting a specialized microenvironment favorable for the establishment and maintenance of stem-cell populations was originally proposed for the hematopoietic system and later applied to the intestinal crypt. A stem-cell niche is a tissue compartment that relies upon external signals that modulate the intrinsic cell regulators for self-renewal, proliferation, differentiation, or cell death. Consistent with the need to maintain an uninterrupted supply of germ cells for spermatogenesis along the complete length of each seminiferous tubule, there is a requirement for an appropriate distribution of the stem cells. The location of stem cells can be traced back to the development of the fetal seminiferous cords, where their precursors, the gonocytes, appear to be randomly placed among the early Sertoli cells. In the postnatal prepubertal testis, the initiation of spermatogenesis and associated growth of seminiferous tubules occurs uniformly throughout the testis. With time, complete cycles of the seminiferous epithelium are established, with fairly precise spatial distribution along the seminiferous tubules, suggesting nonrandom locations of stem cells resting on the basal lamina. Using histologic sections, evidence for the distribution of spermatogonial stem cells in rodent tubules showed their preferential localization close to the vascular-rich intertubular regions, as opposed to neighboring tubules.28 In living mouse tubules with GFP-tagged spermatogonial stem cells, this finding was confirmed and extended to show that spermatogonia derived from these cells formed cell chains that became uniformly distributed along the basal lamina of the tubules.29 If GFP-tagged tubule segments were freed of vessels and interstitial tissues and transplanted into a recipient testis, the association between labeled stem cells and newly-organized intertubular tissue was reestablished in the grafted tubules. Although these studies did not identify a definitive stem-cell niche, the data suggest that those Sertoli cells associated with the nonrandom positions of spermatogonial stem cells have unique functions which remain to be discovered. The relationship between the types of somatic cells and the postulated stem-cell niche is shown in Fig. 136-6.30

FIGURE 136-6. The site of the spermatogonial stem-cell (SSC) niche in the seminiferous epithelium. SSCs are in contact with the basal lamina and are found in the basal compartment of the seminiferous epithelium, below the Sertoli cell tight junctions and surrounded by Sertoli cell cytoplasm. In addition to factors produced by Sertoli cells that regulate SSCs, the nearby peritubular cells and Leydig cells are possible additional sources of molecules that influence SSC functions.
(From Johnston DS, Wright WW, DiCandeloro P et al: Stage-specific gene expression is a fundamental characteristic of rat spermatogenic cells and Sertoli cells, Proc Natl Acad Sci U S A 105:8315–8320, 2008.)
The growth factor glial cell line–derived neurotrophic factor (GDNF) is an essential cytokine for maintenance and proliferation of mouse, rat, hamster, and bull spermatogonial stem cells in vitro; importantly, GDNF is produced by the Sertoli cells and regulated by FSH.31,32 Other cytokines such as basic fibroblast growth factor (bFGF), epidermal growth factor (EGF), and colony-stimulating factor 1 (CSF-1) also appear to augment the role of GDNF in the regulation of stem-cell renewal and proliferation.30,33 Further studies of the genes that govern the fate of spermatogonial stem cells during normal spermatogenesis may provide insight into mechanisms that are disrupted in disordered spermatogenesis that forms the basis of some causes of male infertility.
Type A spermatogonia are the stem cells, or cells that are the precursors of all further-differentiated germ cells and are capable of prolonged or indefinite production of more of their own kind. Primates and humans show dark and pale type A spermatogonia; the former, which are designated as A dark (Ad), have deeply staining, homogeneous, finely granulated chromatin with a central pale-stained area in the nucleus. Pale type A (Ap) spermatogonia exhibit pale-staining nuclei, no central vacuole-type area, and one or two nucleoli associated with the nuclear membrane. Type B spermatogonia show coarse, deeply stained clumps of heterochromatin subjacent to the nuclear membrane, and a central nucleolus.
As a renewing tissue, the seminiferous epithelium has three cell compartments: (1) self-renewing stem cells, (2) cells that via proliferation amplify the numbers of differentiating cells, and (3) a differentiating compartment in which germ cells are committed to mature into spermatozoa. Type Ad spermatogonia rarely divide in the human testis; they serve as reserve stem cells and only divide and transform into type Ap cells after irradiation or cytotoxic treatment.34 Type Ap spermatogonia also function as stem cells by self-renewal and by mitotic proliferation into type B spermatogonia.35 In this sense, Ap cells belong to the amplifying compartment, which also includes type B spermatogonia because the latter divide by mitosis and then progress to become primary spermatocytes.
Spermatocytes belong to the differentiating compartment, and these cells undergo two cell divisions during the process of meiotic maturation and become spermatids destined for transformation into spermatozoa. In humans, the ratio of type Ad, type Ap, and type B spermatogonia and spermatocytes is 1 : 1 : 2 : 4, respectively, and Ad and Ap spermatogonia are arranged in homogeneous groups along the seminiferous tubule36 (see Fig. 136-3). Within these groups, the spermatogonia form pairs of morphologically identical cells, which suggests that their mitotic behavior is “equivalent,” that is, the products of their proliferation are either the same cells or a pair of differentiated spermatogonia.22
The proliferation and differentiation of spermatogonia are regulated by hormones (gonadotropins, androgens [see later]), local growth factors, intercellular paracrine/autocrine factors, and systemic nutrients. They arise from the gonocytes, which are in turn products of the primordial germ cells. Basally located in the seminiferous epithelium, the spermatogonia are partly surrounded by adjacent Sertoli cells, and their contact with the basal lamina places enables the secretory products of peritubular tissues and Leydig cells, hormones, and other factors to influence their function.
Vitamin A is essential for the maintenance of spermatogenesis insofar as vitamin A deficiency in rats and mice results in the arrest of type A spermatogonial proliferation, which is reversed after the administration of either vitamin A or retinoic acid.18 Interleukin 1α produced by Sertoli cells stimulates the proliferation of differentiating spermatogonia in vivo and in vitro in the rat,37 and similar effects have been reported for insulin-like growth factors, also produced by Sertoli cells.38 Spermatogonia express the c-kit receptor transmembrane protein, which promotes proliferation of these cells in association with the receptor ligand termed stem cell factor, produced by Sertoli cells.39 A proto-oncogene, c-kit is associated with the dominant white spotting (W) locus in mice, mutations of which lead to defects in spermatogenesis.40 Stem cell factor is associated with the Steel (Sl) locus in mice, and mutations can cause deficiencies in germ-cell development.41 Stem cell factor and c-kit are also found in the human testis and appear to be abnormally expressed in testicular tissues showing defective spermatogenesis.40 Other factors affecting spermatogonial proliferation include glia-derived neurotropic factor, neurotropin-3, nerve growth factor, epidermal growth factor, platelet-derived growth factor, and follistatin.31,43–46 Mounting evidence indicates that a number of genes on the human Y chromosome (and perhaps some autosomal genes47) are candidates for regulation of spermatogenesis, including the proliferation and/or maturation of spermatogonia.48 Several loci on the long arm of the Y chromosome, Yq, if lost through microdeletions, are associated with oligozoospermia or azoospermia, and some of the genes within these regions are crucial for spermatogenesis. Two genes, DAZ (deleted in azoospermia) and RBM (RNA-binding motif), are present in multiple copies, and if these are deleted, azoospermia or severe oligozoospermia will result.49,50 Both are RNA-binding proteins and are testis specific. In recent studies, the heterochromatic and euchromatic gene sequences of the human Y chromosome have been mapped and used to define the male-specific region (MSY) that makes up 95% of the chromosome’s length and contains specific sequences called amplicons, which are essential for spermatogenesis.50 Gene conversion, that is, nonreciprocal recombination within the Y chromosome itself, is as frequent as reciprocal crossover in autosomes. The MSY euchromatic region is unique to males; approximately 30% of it contains amplicons, whose testis-specific genes are limited or mostly abundant in germ cells, possibly for the purpose of generating unique chromatin configurations. Intra-Y gene conversions may have evolved to conserve testis germ-cell gene functions through evolution.51 As discussed previously, deletions in the MSY region are the most common genetic causes of spermatogenic failure because they arise from homologous rather than nonreciprocal recombinations.52–54 The MSY region contains palindromic sequences that can result in deletions that excise large regions of DNA containing the copies of DAZ and RBM. The identified palindromes can result in smaller deletions, and these small deletions are also associated with low sperm counts.51,54
Opportunities for the study of spermatogonial stem-cell function have become available through the techniques of germ-cell transplantation, cryopreservation, and cell culture.55–58 Successful transplantation of germ cells has been achieved through heterologous or xenologous transfer of cells, in which either rat or hamster germ cells undergo spermatogenic development in recipient mouse testes.59–61 These studies have shown that not only will mouse Sertoli cells support rat or hamster spermatogenesis, but also the rate of transplanted germ-cell development is maintained with the timing characteristic of the donor cells, thus indicating that the rate of germ-cell maturation during spermatogenesis is regulated by germ cells alone. Germ-cell transplantation in primates has also been reported.62
MEIOSIS
Type B spermatogonia enter the prophase of the first meiotic division to form primary spermatocytes. Because of incomplete cytokinesis among dividing spermatogonia, spermatocytes remain connected via cytoplasmic bridges, which persist between all subsequent phases of spermatogenesis until mature spermatids (spermatozoa) are released into the tubule lumen. These bridges contribute to the synchronous development of successive generations of germ cells, which are histologically reflected as interconnected cohorts or families of cells. The arrangement in the seminiferous epithelium of several generations of spermatocytes and spermatids creates morphologically distinct combinations or cell associations in given segments of the seminiferous tubules (see later). Recent studies have shown that spermatids linked by these cytoplasmic bridges can share mRNAs.63
Spermatocytes, classified as primary or secondary, respectively, undergo the first and second meiotic divisions in which the diploid number of chromosomes (44,XY) is reduced to the haploid number (22,X or 22,Y) characteristic of the spermatid. In human spermatogenesis, meiotic maturation is a lengthy process requiring approximately 24 days to progress from preleptotene primary spermatocytes to early round spermatids.64 Preleptotene spermatocytes are the last cells to undertake DNA synthesis resulting in the duplication of each chromosome, the twin copies called sister chromatids. The chromosome number remains diploid, but the DNA content is now 4C, or twice that found in spermatogonia. This event marks the commencement of prophase, which is subdivided into leptotene (thread-type chromosomes), zygotene (paired, homologous, double chromosomes), pachytene (large 20-µm primary spermatocytes with condensed, thickened chromosomes), diplotene (paired chromosomes decondensed and partially separated), and diakinesis (recondensed chromosomes) (Fig. 136-7). Metaphase, anaphase, and telophase follow, when daughter cells separate but remain connected by a cytoplasmic bridge and form two haploid secondary spermatocytes containing 2C DNA. Division II of meiosis proceeds promptly (less than 24 hours), but with no DNA replication, to produce two haploid spermatids of 1C DNA content. Thus, four spermatids may be formed from each primary spermatocyte.

FIGURE 136-7. Sequence of germ cell types in human spermatogenesis commencing with a spermatogonium, progressing through an orderly sequence of cell proliferation and maturation, and terminating in a mature spermatozoon. Explanation of symbols—spermatogonia: type A dark (Ad), A pale (Ap), and B (B); primary spermatocytes: preleptotene (PL), leptotene (L), zygotene (Z), pachytene (P), and meiotic division (M); secondary spermatocyte (II), spermatids (Sa, Sb1, Sb2, Sc, Sd1, Sd2); residual body (RB).
Pairing, or synapsis, of maternal and paternal chromosome homologues becomes evident during zygotene and is completed in pachytene, in which close apposition of the entire length of each homologue pair is maintained by a long, ladder-like core of proteins termed the synaptonemal complex. Genes encoding synaptonemal complex proteins (SCP1) and chromosomal core proteins (COR1) are unique to meiosis. Heat shock protein (HSP70-2) synthesis occurs after its transcription in leptotene-zygotene spermatocytes and is required for desynapsis of synaptonemal complexes and completion of meiosis I.65,66 Pairing contributes to genetic reassortment in gametes by exchange of DNA sequences between maternal and paternal homologues through genetic recombination. This process takes place when the aligned chromosomes in pachytene cross over at locations called chiasmata and requires endogenously induced breaking and ligation of DNA, mediated by proteins that perform error-free repair of double-strand breaks. These proteins are topoisomerases that are strongly induced in midphase primary spermatocytes and conserved from yeast to humans.67 The random distribution of paternal and maternal sets of chromosomes produced by meiosis I could form more than 8 million genetically different gametes, that is, 2n different gametes, where n = 23, the haploid number of chromosomes. Genetic recombination greatly increases the number of variants that can occur in gametes from an individual, based on a common genetic program inherited from their biological ancestors. Small amounts of DNA synthesis can occur during zygotene, possibly involved in the alignment of homologues during the establishment of synapsis, and during pachytene,68 probably associated with DNA repair during genetic recombination.
Spermatocytes engage in RNA synthesis, but a proportion of meiotic transcripts are not released from the nucleus to the cytoplasm until diplotene and constitute a pool of stable mRNA that is translated postmeiotically in spermatids.69 Some of these transcripts provide for enzymes such as lactate dehydrogenase and phosphoglycerate kinase, which are essential for sperm glycolysis, and others encode some proteins used in assembly of the sperm tail during spermiogenesis.
SPERMIOGENESIS
The haploid spermatids are spherical, approximately 8 µm in diameter, and located in the inner third of the seminiferous epithelium. There is no further cell division in spermatogenesis. Because of incomplete cytokinesis in renewing spermatogonia, numerous spermatids remain connected by cytoplasmic bridges and may contribute to their synchronized development in seminiferous tubules. Spermiogenesis refers to the complex transformation of round spermatids into spermatozoa, a process requiring 24 days in humans (Fig. 136-8). Maturation of spermatids has been described extensively in morphologic studies,6,16,70 and molecular biology techniques provide insights into the regulation of gene expression during spermiogenesis.80–84

FIGURE 136-8. Spermiogenesis in the human testis as noted by electron microscopy. Steps Sa and Sb1 and Sb2 correspond to the Golgi and cap phases of cell development, Sb2 and Sc to the acrosome phase, and Sd to the maturation phase. A, Acrosome or acrosomic vesicle; Af, developing axial filament; An, annulus; Ax, axoneme; C, centrioles; Fs, fibrous sheath; G, Golgi complex; M, mitochondria; Mn, manchette; Mp, middle piece; Mt, microtubules in spindle-shaped body; N, nucleus; Ne, redundant nuclear envelope; Nk, neck; Pp, principal piece.
(From Kerr JB: Functional cytology of the human testis, Baillieres Clin Endocrinol Metab 6:235–250, 1992.)
Each spermatozoon has a head and tail. The head of the human spermatozoon is spatulate in form, flattened and somewhat pear shaped, and contains the condensed nucleus. In rodents, the sperm have a falciform head. The coverings of the spermatid head and the microtubules in the developing tail region are thought to influence nuclear shaping during spermiogenesis. A membrane-bound thin cap, the acrosome, extends from the tip or leading edge of the head and covers approximately 60% of the sperm head. A perinuclear theca attaches the acrosome to the nucleus, and a plasma membrane encloses the entire head.
The tail, also delimited by a plasma membrane, consists of four components: the connecting piece or neck, the middle piece, the principal piece, and the end piece. The neck, which articulates with the head, resembles a hollow cone, the base of which is associated with the caudal pole of the nucleus. The sides of the cone consist of nine cross-striated columns continuous with the nine outer dense fibers (ODFs), cytoskeletal elements of the tail. In the core of the neck, the remnant of the distal centriole anchors the tail to the sperm head and also gives rise to the axial filament, or axoneme, of the tail (nine + two arrangement of microtubules). The companion proximal centriole, which remains intact, is also lodged within the cone, and its axis lies at 90 degrees to the axoneme (Fig. 136-9).

FIGURE 136-9. A diagrammatic representation of a cross-section of a typical axoneme is shown, with each of the components being critical for the generation of normal sperm motility.
Extending from the neck, the middle piece (∼5 µm long) includes the central axoneme, which continues to the end of the tail; the ODFs, which encircle the axoneme; and the sheath of mitochondria, which lies external to the ODFs and terminates at the annulus, a ringlike structure beyond which is the principal piece. The latter (∼45 µm long) consists of seven tapering ODFs, ODF-3 and ODF-8 being replaced by longitudinal columns of the fibrous sheath (FS). Specialized structures run circumferentially around the ODFs and join the longitudinal columns. These structures are known as the transverse ribs. Tapering toward its end, the principal piece is devoid of ODFs and the FS and forms the end piece, in which the axoneme terminates as disorganized microtubules.
Based on structural alterations of the nucleus and acrosome (see Fig. 136-8), spermiogenesis may be subdivided into the Golgi, cap, acrosome, and maturation phases.16,70 In summary, during the first two phases, the Golgi complex contributes to formation of the acrosome (marking the cranial pole of the nucleus), which flattens and progressively covers the anterior aspect of the sperm head. At the opposite pole of the nucleus, the pair of centrioles that have commenced formation of the axoneme then lodge in a shallow fossa in the nucleus. In the acrosome phase, the nuclear chromatin begins to condense, and with elongation and remodeling of the nucleus, the species-specific shape of the sperm head is developed. Elongation of the caudal cytoplasm is associated with development of the middle and then the principal piece to form the flagellum. During the maturation phase, most of the spermatid cytoplasm becomes separated from the head and tail and forms several globular masses of residual cytoplasm termed residual bodies. Disengagement of the spermatozoa from the seminiferous epithelium at spermiation is marked by retention and engulfment of residual bodies by the Sertoli cells, which dispose of them by phagocytosis.
The regulatory mechanisms associated with spermiogenesis are not yet well understood and represent a combination of processes in which the microenvironment of the seminiferous epithelium (i.e., the extrinsic biological actions of hormones, growth factors, and biochemical activities of the Sertoli cell) is a corequisite that modulates gene expression and the synthetic and metabolic activities intrinsic to the developing spermatids. Numerous studies of the intrinsic processes regulating gene expression in spermiogenesis are focusing on changes in transcription/translation as the nucleus and its chromatin undergo condensation, as well as mechanisms contributing to formation of the tail.72,73
During spermiogenesis, the spermatid nucleus is dramatically reorganized in shape and size and degree of compaction of the chromatin. The DNA in sperm occupies only approximately 5% of the volume of DNA in somatic cells.74 The basic proteins, the nucleosomal histones of the spermatocytes, are replaced by intermediate proteins (transition proteins) and protamines, leading to an extreme condensation of chromatin.
The commencement of spermiogenesis is associated with a massive wave of transcriptional activity involving the activation of a variety of postmeiotic genes in haploid cells.75 However, translational control of gene expression is important because as the chromatin condenses during the second phase of spermiogenesis, nuclear transcription activities are progressively shut down. The silencing of transcription is achieved by the replacement of histones by protamines, such that the spermatids are almost devoid of histones. The somatic-type histones are thought to be acetylated, causing disassociation with DNA followed by ubiquitination, allowing their removal in favor of transition proteins (TP1, TP2).76 The latter enhance DNA compaction and may be involved in the repair of DNA breaks that arise during chromatin remodeling.77 However, TP1 and TP2 knock-out mice are fertile, suggesting that these proteins are redundant and that histone replacement and chromatin compaction may proceed independently of transition protein formation.78,79 The last highly basic proteins associated with the DNA are the protamines (HP1-4 in man), which are transcribed early, but the resulting mRNA is stored (for several days or more) before translation into protein.69,72,73 These stable mRNAs and possibly numerous others (e.g., those produced during meiotic prophase with translational repression until spermiogenesis) are thought to be stored or bound to specific RNA-binding proteins until translation is required.80,81
A structure known as the chromatoid body appears in spermatids as a single, lobulated, perinuclear granule that migrates to form a ring with the annulus around the developing flagellum. Recent data confirm the concept that this structure is involved in storing and processing of haploid transcripts82 and, through the kinesin motor protein KIF17b, is mobile and implicated in RNA metabolism. Their findings link the chromatoid body in microRNA-dependent regulation of specific mRNAs in spermiogenesis.
Premature translation of protamine mRNA in transgenic mice causes precocious condensation of nuclear DNA, with arrest of final spermiogenesis and infertility.83 Protamines organize the spermatid genome into very specialized doughnut-shaped chromatin components that are quite distinct from the usual architecture of the nucleosomes,84 which may be necessary for specific translation activities after fertilization.
The acrosome is a modified secretory granule with distinct regional molecular composition and function associated with its membrane and matrix. It has two biochemical components: a soluble protein compartment and an insoluble acrosomal matrix. Hyaluronidase and dipeptidyl peptidase are found in the soluble phase, whereas proacrosin and proacrosin-binding protein occur in the latter.85 Soluble proteins are released during acrosome exocytosis, but the matrix proteins remain with the sperm, mechanisms which may regulate the acrosome reaction and sperm–zona pellucida interaction. Hydrolytic enzymes are bound to the protein matrix, and in the course of fertilization, acrosomal hydrolases are released during the acrosome reaction to allow sperm penetration of the zona surface. The outer acrosomal membrane–associated matrix complex proteins show sequence homology between bovine, primate, human, and mouse spermatozoa86 and may localize a hydrolase pool at the sperm-zona binding site, maintaining attachment of the sperm to the zona pellucida. Men with failure of acrosome formation are infertile, and their sperm take on a globular shape, the condition being termed globozoospermia. Mice with a targeted disruption of casein kinase II, a catalytic subunit gene, show the absence of the acrosome and globozoospermia.87
Details of the genetic basis and molecular biology of the assembly and function of the sperm tail are emerging and suggest that in addition to providing a structural framework for motility, they may provide mechanisms by which a spatial distribution of proteins and enzymes facilitate the complex biochemistry of flagellar movement.
The core axoneme comprising two central single microtubules and nine peripheral doublet microtubules is conserved in cilia and flagella from algae to humans. Genes encoding key components essential for motility have been defined, and mutations in these genes constitute a clear genetic basis for infertility and respiratory dysfunction, known as ciliary dyskinesia (see review for details).88 Others may account for a minor yet significant proportion of male infertility.89
Some genes and their encoded proteins that comprise the neck, the ODFs, and the FS have been identified.71,90–92 The precise function of ODFs is unclear, but their elastic properties and tensile strength may be integral components of normal flagellar motility. Expression of genes encoding some of these proteins occurs in early round spermatids and reaches peak levels during the acrosome phase. Some ODF proteins appear to be stored in granulated bodies in the spermatid cytoplasm before their assembly in a proximal-to-distal direction along the axoneme.71,90 Immunogold-labeling studies with anti-ODF-27 and anti-ODF-84 antibodies have shown localization to the neck of the tail, thus confirming that the segmental columns and basal plate contain cytoskeleton-type proteins similar to those in the flagellum.90,91
Immunocytochemistry has shown that FS proteins are assembled in a distal-to-proximal direction along the axoneme, eventually meeting and overlapping the ODF assembly within the periaxonemal cytoplasmic compartment.71 Unlike the association of ODFs within cytoplasmic granular bodies, FS proteins are randomly distributed in the caudal spermatid cytoplasm and then targeted directly along the axoneme to their site of assembly.
A junctional complex structurally links the spermatids to the plasma membrane of Sertoli cells and specifically flanks the developing acrosome region of elongating spermatids to regions of ectoplasmic specialization (ES) of the Sertoli cell.93 These ESs resemble half of the unique inter–Sertoli cell junctional specializations that form the blood-testis barrier located in the basal region of the seminiferous epithelium (see later). The adhesive properties of ESs facing elongating spermatids are attributable to a complex association of cytoskeletal proteins, including actin, vinculin, integrins, and α-actinin. Ectoplasmic specializations are thought to orient spermatids within the seminiferous epithelium; for example, when step 7 round spermatids in the rat rotate during step 8 of spermiogenesis such that the cranial pole with the developing acrosome faces basally, the caudal cytoplasm then points apically.6 Adhesion at the interface of the ES and acrosome, shown to be androgen dependent, is also necessary to prevent spermatid detachment from the Sertoli cells.94 In the immature testis, acquisition of binding between Sertoli cell ESs and post–step 8 spermatids may be dependent on a “primary effect” of follicle-stimulating hormone (FSH)-stimulated maturation of Sertoli cells.95
Studies of the developmental competence of spermatids with respect to their capacity to fertilize a mature oocyte and lead to the production of normal, fertile offspring have shown that this ability is achieved early in spermiogenesis. Round spermatid nuclei from mice, with or without cytoplasm, can initiate normal embryo development when electrofused with or injected into oocytes,96,97 and cryopreserved round spermatids have been used to achieve the same result.98 These findings indicate that imprinting of parts of the genome necessary for normal embryonic development is accomplished during the initial phases of spermiogenesis.
GERM-CELL DEGENERATION
Germ-cell degeneration is common during testicular development and in adults and is a major factor in determining the efficiency of spermatogenesis in terms of the theoretic versus the observed yield of mature spermatozoa.18,99 Excessive germ-cell degeneration may arise either in response to external or extratesticular influences (e.g., seasonality, nutrition, therapeutic drugs, environmental agents, infection) or as a result of intrinsic dysfunction of spermatogenesis, the details of which are largely unknown. Depending on the species, as much as 75% of the expected sperm yield may be lost in normal spermatogenesis. Degeneration with death of germ cells is attributable to apoptosis, in which activation or suppression of particular genes dictates a cell death or survival pathway. Apoptotic cells occur in most tissues in which a balance between cell proliferation and elimination is required for normal growth and/or maintenance of the cellular population. In contrast to necrosis, apoptotic cells fragment and form several or numerous condensed bodies that include the pyknotic nucleus, but these bodies do not lyse, instead being phagocytosed by neighboring cells, and in the testis, the Sertoli cells rapidly dispose of these elements. Apoptosis therefore does not normally initiate an inflammatory response. In mice, a growing number of genes have been identified that, if deleted or disrupted, lead to germ-cell apoptosis at various phases of spermatogenesis, and most of these genetically altered mice are infertile.99 A range of proteins termed the Bcl-2 family act to either promote (Bax, Bak, Bcl-xs, Bad proteins) or prevent (Bcl-2, Bcl-xL, Bcl-w, Mcl, Al proteins) apoptosis.100,101 These proteins form homodimers or heterodimers with each other, and the balance between them determines whether a cell will enter the apoptotic pathway. The expression of these proteins are developmentally controlled, and disruption of the genes encoding these proteins results in disordered spermatogenesis, often through mechanisms that alter the Sertoli cell–germ cell ratios.101,102
Spontaneous germ-cell apoptosis occurs in all phases of human spermatogenesis, and its incidence varies with ethnic background.5 Numerous studies in laboratory animals103,104 and in humans105,106 have shown that this process is hormonally dependent (gonadotropin and androgen deprivation) and activated in response to heat stress, radiation, antimetabolic agents, cytoskeletal disrupters, cytotoxic agents, and tumor-inducing drugs. The precise causes and significance of germ-cell death during spermatogenesis remain unclear, but the emerging details of apoptosis in the testis are consistent with the following speculations107: (1) dividing germ cells are deleted if they harbor nonrepaired DNA, (2) spermatocytes are eliminated if after chromosomal exchange in pachytene they contain faulty DNA, and (3) spermatids (or any germ cell) will degenerate if they are overproduced and therefore inadequately supported by Sertoli cells. Maintenance of normal function and numbers of germ cells by control of their survival versus death may be a key factor in the complex organization and synchronization of spermatogenesis.
GENETIC DEFECTS AND SPERMATOGENESIS
Although a thorough description of how spermatogenesis is affected by specific gene activity is beyond the scope of this chapter, it has been proposed that in approximately 60% of men with idiopathic spermatogenic disruption, a genetic cause is suspected.47,108 More than 100 gene mutations can cause spermatogenic disruption and fertility defects in mice.109,110 Random mutations in mice have been generated by the use of chemical mutagens such as ethylnitrosourea or, in the case of embryonic stem cells, with ethylmethanesulfonate,111 and the mutations resulting in spermatogenic disruption are being identified and providing novel concepts as to the genetic regulation of spermatogenesis.
GERM-CELL ASSOCIATIONS AND SPERMATOGENIC CYCLE
Coordinated and synchronous development of germ cells occurs within the seminiferous tubule. Cell associations are defined combinations of germ cells present at any moment in a given site of the seminiferous epithelium, an example of which is seen in Fig. 136-4. For particular species, these associations are classified by Roman numerals into stages, such as I to XIV for rat, I to XII for the guinea pig, and I to VI for humans.6,112 If it were possible to observe stages for prolonged periods in living seminiferous tubules, the morphology of each stage in a fixed tubular location would gradually transform into the stage with the structural features of the next Roman numeral. On reaching the highest numbered stage applicable, the germ-cell association transforms into stage I and continues thereafter to proceed through successive stages. The mechanisms underlying this synchronous development are (1) controlled proliferation of spermatogonial stem cells and their production of young spermatocytes, (2) fixed time intervals associated with meiotic maturation of spermatocytes into spermatids and the latter into spermatozoa, (3) coordinated development of cohorts of germ cells linked by cytoplasmic bridges, and (4) interactions between all germ cells and Sertoli cells, which occur in response to transduction of hormonal stimulation of the seminiferous epithelium. In most mammals and nonhuman primates, stages stretch along seminiferous tubules for as long as several millimeters, and when isolated tubules are examined by low-magnification transillumination microscopy, consecutive density patterns may be observed and reflect changes in germ-cell number, position, and density. When an identical stage reappears along the length of a tubule, the distance between them is defined as the wave of the seminiferous epithelium. A complete sequence of stages (14 in the rat) requires passage of a species-specific interval (in days) referred to as one cycle of the seminiferous epithelium (12 days in rats and 16 days in humans). Commencing with the proliferation of a spermatogonium, completion of spermatogenesis requires that the germ cell passes through four cycles as it is transformed into a spermatozoon. The orderly, segmental sequence of stages is noted in cross-sections of tubules in which the same stage of the seminiferous cycle occupies the entire tubule (see Fig. 136-4), but in humans and some primates (baboon, crab-eating macaque, orangutan, chimpanzee),18,22,112 several stages are present in transversely sectioned tubules (see Fig. 136-4). In some selected examples of human seminiferous tubules, the arrangement of developing germ cells follows a series of overlapping helical strips (Fig. 136-10) that gyrate toward the tubule lumen112 (Fig. 136-11). Other studies have shown that this helical pattern is the exception rather than the rule, inasmuch as a partial wave in humans appears to be a random occurrence.113 The architectural composition of stages within human seminiferous tubules varies with the level of efficiency of spermatogenesis. Men with high daily sperm production per gram of testis parenchyma (7.5 ± 0.2 × 106) show more stages per cross-sectional tubule than men with lower sperm production (3.6 ± 0.3 × 106). Incompleteness of the wave in the human testis may be a variant of the same phenomenon in the rodent testis, in which most (80%) tubules show localized site reversal of the numerical order of stages along the tubules, and as many as nine of these modulations have been noted in a single entire tubule.112–116

FIGURE 136-10. Helical arrangement of the development of primary spermatocytes of the human testis (leptotene [L], zygotene [Z], pachytene [P]) drawn as strips that overlap and gyrate toward the tubule lumen. This pattern, when apparent, occupies only small segments of the seminiferous tubule; otherwise, the stages of spermatogenesis (I-VI) occur randomly along the tubules.

FIGURE 136-11. Representation of stages I through VI of the spermatogenic cycle of the human testis, with their complement of germ cells arranged to show how the sequence of development corresponds to the geometry of spirals gyrating conically toward the lumen. In actual tubules, usually two to four stages per cross-section are seen, some of which are located at slightly more superficial or deeper levels along the seminiferous tubule. Symbols are as defined in Figure 136-4.
(From Kerr JB: Functional cytology of the human testis, Baillieres Clin Endocrinol Metab 6:235–250, 1992.)
How the orderly arrangement of germ-cell associations along the seminiferous tubules is established at the time of initiation of spermatogenesis is unknown, but it is possibly regulated by local interactions between the maturing Sertoli cells and the intertubular tissue. Support for the role of Sertoli cells has been demonstrated by differential gene expression among immature Sertoli cells in relation to the numbers and types of germ cells with which they associate.117 Differentiating spermatogonial cells derived from Ngn3-negative gonocytes arise in specific segments of seminiferous cords/early tubules that express high levels of galectin 1, a carbohydrate binding protein expressed by Sertoli cells.117 The switch from initiation to steady-state spermatogenesis in the pubertal testis generates differentiating spermatogonia from self-renewing Asingle stem cells, and this phase occurs in tubule segments with high galectin 1 expression, that is, stages IX-X of the spermatogenic cycle. Galectin 1 transcripts are detectable in localized segments of seminiferous cords in E18 mouse embryos prior to morphologically recognizable heterogeneity among the germ cells and is retained with similar cyclic patterns of gene expression in the cords of germ cell–deficient mutant mice.117 Together, these findings point to the requirement of Sertoli cells for a major role in establishing the spatiotemporal patterning of the seminiferous epithelium.
Regulation of the Spermatogenic Cycle
The duration of the cycle of the seminiferous epithelium is defined as the time interval needed for one complete series of germ-cell associations to appear at a fixed point within the adult seminiferous tubule.118 The length in days of the spermatogenic cycle is constant for a given species (e.g., 8 days in mouse, 12 days in rat, 16 days in man). The total duration of spermatogenesis requires approximately 4.5 cycles in mammals; for the mouse this is 35 days, in the rat, 50 days, and in the human about 70 days. Evidence for the germ cells as the regulators of the duration of spermatogenesis has come from xenografting studies whereby germ cells obtained from rats or hamsters were transplanted into recipient mouse testes. The donor germ cells establish spermatogenesis with a duration of the seminiferous cycle similar to that in situ.59,60 In contrast, grafts of immature monkey or neonatal pig testis tissue implanted ectopically into host mice result in accelerated testicular maturation and precocious spermatogenesis in the transplants in comparison to the expected time required to achieve full spermatogenesis.119,120 These results suggested that the shorter time to full spermatogenesis was due to (1) accelerated maturation of the Sertoli and Leydig cells and/or (2) alteration of the length of the spermatogenic cycle. Using BrdU-labeling of dividing cells, a reexamination of spermatogenesis in newborn ovine and porcine testis fragments transplanted ectopically into mice showed that the length of the spermatogenic cycles in xenografts was conserved and similar to their durations in situ.121 It was concluded that precocious spermatogenesis observed in grafted immature seminiferous tubules is a consequence of accelerated maturation of the testicular somatic cells, probably driven by the activity of the host’s hypothalamic-pituitary axis.
The factors that govern entry of germ cells into spermatogenesis and their progression within the spermatogenic cycle are mostly unknown and probably are represented by hundreds if not thousands of testis-specific proteins that maintain a repeating program of germ-cell proliferations, maturation, and morphogenesis. Attempts to identify specific gene expression associated with selected stages of spermatogenesis have had limited success, owing to difficulties in deciding which gene products are constitutive and which are preferentially involved with one or more of the many processes that shape the development of germ cells.122 Microarray analysis of nearly 400 gene transcripts unique to the rat testis have been described,123 and over 1200 transcripts show significant (fourfold) variations closely synchronized across the 14 stages of spermatogenesis.
POSTNATAL GROWTH OF THE HUMAN TESTIS
Quantitative assessment of seminiferous tubule growth is available for ages 0 to 18 years.124,125 Testis volume increases significantly from 0 to 10 years: from 1.1 mL (median value of paired testes) in the 0- to 1-year age group to 3 mL at ages 5 to 10 years. At 14 to 18 years, median paired testis volume is greatly increased to 23 mL and continues to increase to 40 mL in an adult group aged 18 to 50 years (Fig. 136-12). Seminiferous tubule length per testis also increases with age, showing a biphasic pattern in the pre- and postpubertal age ranges and reaching a median length of 600 m. The latter figure varies widely between individuals, in some cases reaching in excess of 1000 m. Until the age of 14 years, the tubule diameter does not appreciably increase from birth (50 to 60 µm), but thereafter expands to approximately 130 µm in the 14- to 18-year age range and in adults is approximately 200 µm. Other studies from birth to 10 years of age also report no increase in tubule diameter, although the slightly larger size of 70 to 80 µm probably reflects postmortem change before tissue fixation.126 Growth in the length but not the diameter of the seminiferous tubules in the prepubertal period is consistent with the fact that the testis in childhood is not a quiescent organ but shows a significant increase in the total number of germ cells. From 1 to 10 years of age, the total number of germ cells per testis is estimated to increase from 13 to 83 million and grow exponentially after this stage.124 Much of this cellular growth is attributable to proliferation of the spermatogonia that populate the growing seminiferous tubules by forming colonies that migrate and expand along the length of the tubules.124,126,127

FIGURE 136-12. Growth in testis volume and seminiferous tubule length in 50 boys from ages 0 to 18 years. Tissue samples obtained postmortem were fixed and processed in paraffin. Stained sections were analyzed using quantitative histologic methods.
(Data based on Muller J, Skakkebæk NE: Quantification of germ cells and seminiferous tubules by stereological examination of testicles from 50 boys who suffered from sudden death, Int J Androl 6:143–156, 1983.)
Sertoli Cells
Sertoli cells are the only somatic, nondividing cell population within the seminiferous tubules, where they occupy as much as 25% of the volume of the seminiferous epithelium. Because they extend from the basal lamina to the tubule lumen, each of these cells partly or completely surrounds several dozen or more germ cells, for which they provide structural and biochemical support together with local immunologic protection. The literature on Sertoli cells is considerable and detailed6,15 and provides some insight into precisely how Sertoli cells support the process of spermatogenesis.
SHAPE AND DISTRIBUTION
Sertoli cells resemble the shape of a tree in that they are tall columnar cells resting on the basement membrane and extending lateral and apical cytoplasmic branches from the body or trunk of the cell that overarch the spermatogonia and interdigitate and surround all other germ cells (see Fig. 136-3). By providing physical support to the germ cells, the Sertoli cell must maintain a degree of rigidity while retaining the capacity to change shape in synchrony with the division, enlargement, and metamorphic alterations of germ cells. Attainment of quantitatively normal spermatogenesis in an adult depends on an adequate number of Sertoli cells,128,129 which is achieved by their proliferative activity during growth of the fetal and postnatal testis and is dependent on FSH and activin stimulation.130–133 Further proliferation takes place during puberty in mammals, in which birth and puberty are significantly separated temporally.150
Quantitative histologic studies of the human testis have indicated that Sertoli cell numbers may increase until approximately 15 years of age.134 At its base, each Sertoli cell is in contact with five or six adjacent Sertoli cells, and each cell supports as many as 50 germ cells throughout the depth of the seminiferous epithelium.15,135
BLOOD-TESTIS BARRIER
The blood-testis barrier is a structural and physiologic compartment created by inter–Sertoli cell tight junctions located above the basally positioned spermatogonia and the earliest germ cells that develop from them, the leptotene/zygotene primary spermatocytes (Fig. 136-13 and seen in Fig. 136-2). The junctions form the blood-testis barrier and are stabilized by a large number of component proteins,136 including subsurface actin filaments, actin-binding protein such as espin, and smooth membranous cisternae.135 Their unusual position at the base of the seminiferous epithelium is known to subdivide the epithelium into basal and adluminal regions that partition young and more mature germ cells into two distinct anatomic and functional compartments. A basal epithelial compartment separating these germ cells from more mature germ-cell types is believed to be essential for (1) excluding postmeiotic germ cells from immunologic challenge arising from the intertubular tissues, (2) maintenance of the basal stem-cell niche, (3) creating a barrier against paracellular fluid and macromolecular flow into the epithelium, and (4) selective exposure of basal germ cells to regulatory factors originating from Leydig cells and peritubular cells.

FIGURE 136-13. Diagram illustrates the manner in which the cell membranes of adjacent Sertoli cells form the tight occluding junctions that constitute the blood-testis barrier. Also illustrated is how developing germ cells move from the basal to the adluminal compartment of the testis. Passage of tracers through the intercellular space is illustrated (arrows).
How then do the early primary spermatocytes migrate from the basal to the adluminal compartment, given the location of the tight junctions? Using lanthanum salts as an extracellular tracer molecule, a partial answer emerged when it was suggested that the passage of a basal spermatocyte into the adluminal compartment was accompanied by a transient intermediate compartment whereby tight junctions were disassembled/open above and reformed below a migratory germ cell.137 This theory has been confirmed both in vivo and in vitro.138,139 Disruption of the adluminal tight junctions is mediated by release of transforming growth factor β2 [TGF-β2] from Sertoli cells and/or transiting spermatocytes. The free tight-junction proteins, such as occludin, are sequestered to lysosomes for disposal or to endosomes. In contrast, testosterone acts to recycle blood-testis barrier proteins back to the Sertoli cell surface for reassembly into new tight junctions located below a migrating germ cell. Thus testosterone and a cytokine act together to differentially regulate and coordinate the dynamics of the tight junctions, maintaining the integrity of the blood-testis barrier, yet allowing germ cells to cross the barrier and continue with meiotic maturation.
It is notable that these events do not occur in isolation within the seminiferous epithelium but are synchronized with the release of mature spermatids from the opposite domain of the Sertoli cell that faces the tubule lumen. The proteins comprising the tight-junction complexes are represented in part in the ectoplasmic specializations (similar to hemitight junctions) that provide for attachment between spermatids and Sertoli-cell plasma membranes. Disengagement of the ectoplasmic specialization enables spermiation and the release from the disassembled ectoplasmic specialization of fragments of laminin, a protein forming a laminin-integrin adhesion complex at the this site.140 Laminin fragments have been shown to destabilize the blood-testis barrier by reducing the levels of occludin in the tight junctions.139 It is hypothesized that ectoplasmic specialization-mediated spermiation and blood-testis barrier restructuring comprise a Sertoli cell autocrine axis to coordinate replacement of new germ cells entering the adluminal compartment, since the most mature generation of germ cells is lost through spermiation.
The blood-testis barrier exists in all animals6 and is formed during the initiation of spermatogenesis in response to gonadotropic stimulation141 and the appearance of zygotene-pachytene primary spermatocytes. In the developing human testis, junctional specializations between Sertoli cells are absent until 8 years of age, but in the early phase of puberty (11 to 13 years), the typical inter–Sertoli cell junctions are assembled.142 These junctions are impermeable to lanthanum penetration and coincide with the appearance from 12 years of age of increasing numbers of spermatocytes, consistent with the establishment of basal and adluminal epithelial compartments.
PLASTICITY AND LINKS WITH GERM CELLS
Sertoli cells exhibit an elaborate and dynamic cytoskeleton that serves to maintain the columnar shape of the cell, determine the intracellular distribution of organelles, and provide intercellular adhesion with germ cells. The supranuclear regions of the Sertoli cell cytoplasm are supplied with microtubules (chiefly α-tubulin) and the motor proteins dynein and kinesin, which are especially abundant in the crypts surrounding elongating spermatids.143,144 Together with intermediate filaments, these cytoskeletal elements are believed to influence the shape and position of the germ cells in concert with the spermatogenic cycle. A further component of the cytoskeleton of Sertoli cells is evident at the interface between the plasma membranes of Sertoli cells and germ cells and is identified as an ES. The ES appears to be identical to half of the inter–Sertoli cell tight junction and consists of a dense mat of actin filaments sandwiched between the plasma membrane and a cistern of smooth endoplasmic reticulum.135,145 Only rarely associated with zygotene primary spermatocytes, they are more frequently observed facing pachytene primary spermatocytes and always occur in association with round and elongating spermatids and form a mantle around the heads of the latter germ cells.7 The presence of vinculin within these ESs146 suggests that Sertoli cells adhere to the plasma membrane of germ cells and thus play a role in determining the shape of the elongating sperm head and in adjusting the orientation of the germ cells within the seminiferous epithelium. Translocation of spermatids within the deep recesses created by the Sertoli cells is shown in Fig. 136-14.

FIGURE 136-14. Scheme of spermatid translocation hypothesis shows relationship between ectoplasmic specializations of germ cells and their transport along Sertoli cell microtubules via motor proteins. After spermiation, ectoplasmic specializations disassemble and associate with spermatids. With adluminal migration of early spermatocytes from the basal to the adluminal compartment, the Sertoli ectoplasmic specialization is degraded then reassembled to seal the inter–Sertoli cell tight junctions.
(Redrawn from Vogl AW, Pfeiffer DC, Mulholland D et al: Unique and multifunctional adhesion junctions in the testis: ectoplasmic specializations, Arch Histol Cytol 63:1–15, 2000.)
The Sertoli cell microtubular system is especially abundant and may act as an intracellular transport apparatus that moves germ cells by coupling the motor proteins to an intercellular junction in the form of ESs.144 It is thought that the ES-spermatid association allows microtubule motors within the columnar Sertoli cell cytoplasm to direct the movement of elongating spermatids initially in a basal direction via the microtubule-associated protein kinesin. With further development of the spermatid, particularly as the axoneme lengthens, the microtubule motor protein dynein then translocates the spermatid in the reverse direction toward the apical region of the Sertoli cell. Why it is necessary for elongating spermatids to penetrate into deep Sertoli cell crypts is unknown, but this close association with the surrounding Sertoli cell cytoplasm may assist with stabilization of the long (50 µm) spermatid tail during its assembly and restructure of its ultimately redundant cytoplasm.
PHAGOCYTOSIS, ENDOCYTOSIS, AND SECRETION
Sertoli cells phagocytose the excess residual cytoplasm of spermatids shed at spermiation and eliminate degenerating germ cells, which is a naturally occurring phenomenon during spermatogenesis. The residual bodies fuse with endosomes and lysosomes and migrate toward the base of the Sertoli cell.21 Lysosomal numbers and enzymatic activities vary throughout the spermatogenic cycle147,148 and serve to dispose of degenerative germ-cell products. The endocytosis of tracers such as ferritin has suggested that the lysosomal system also participates in the reduction of excess plasma membrane and absorption of luminal fluid through the apical cytoplasm.147 Sertoli cells show receptor-mediated endocytosis of macromolecules via their apex and base,149 and recent in vivo and in vitro studies have demonstrated this to be the mechanism by which the Sertoli cells deliver iron to germ cells from the serum.150 The Sertoli cells in adult males do not divide, and consequently the complement of Sertoli cells is established at the conclusion of puberty.
Intertubular Tissue
ORGANIZATION
The region between the seminiferous tubules, termed the intertubular or interstitial tissue, consists of loose connective tissue, the cellular and extracellular components of which are qualitatively similar in the mammalian testis, although its quantitative composition is species specific.6 The relative proportion of Leydig cells, which synthesize and secrete androgens (chiefly testosterone), varies between species, usually occupying only 10% to 20% of the intertubular tissue (rat, guinea pig, ram, human).6 Blood vessels occur randomly throughout the intertubular tissue, and capillaries are nonfenestrated. Lymphatic vessels and large lymphatic sinusoids are seen in several rodent species (mouse, rat, guinea pig), in contrast to the human testis, in which these vessels exist only in the main connective-tissue septa extending inward from the tunica albuginea.151 Fibroblasts and macrophages are also found in the intertubular tissue, whereas lymphocytes and mast cells are irregularly distributed and occur more commonly in the region of the tunica vasculosa.
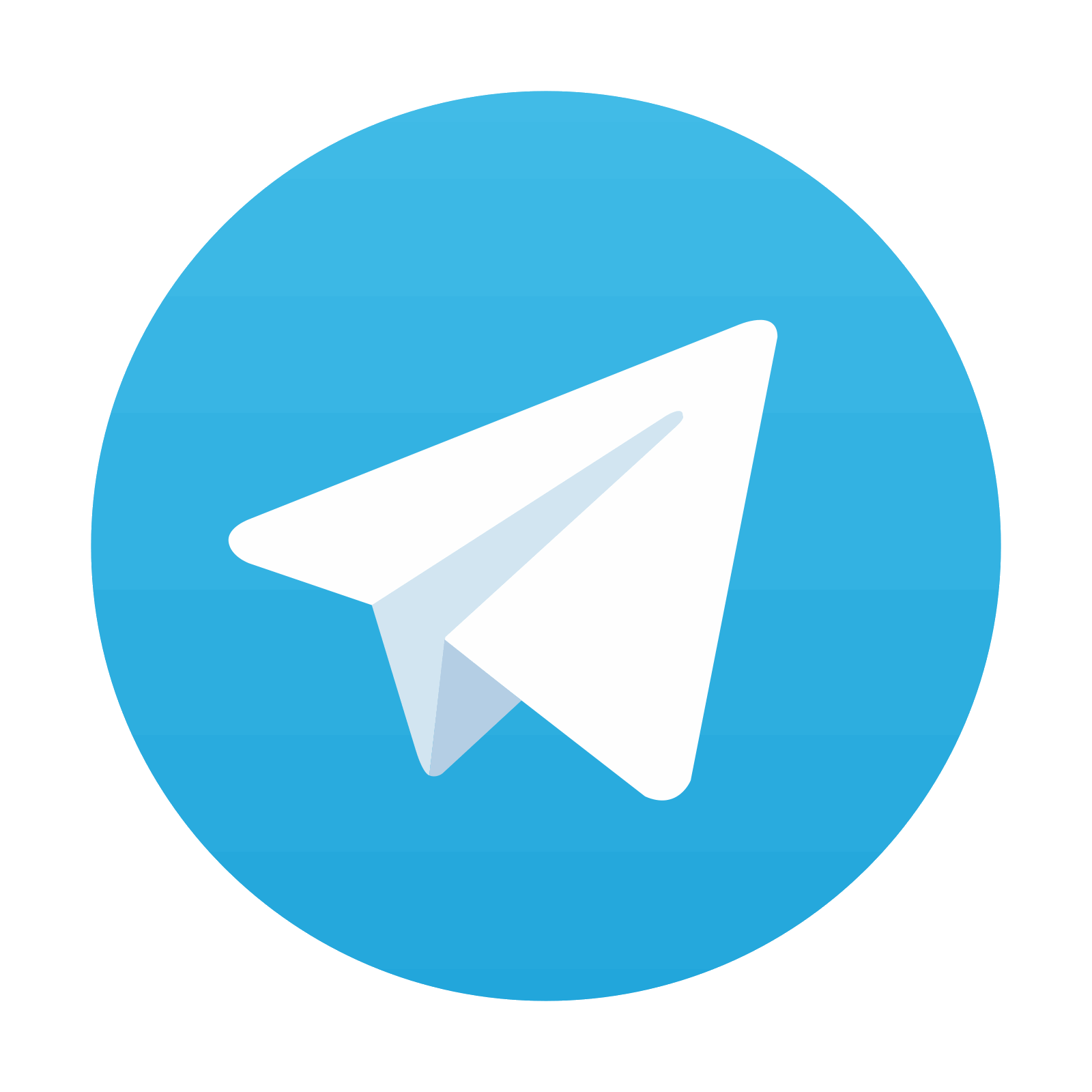
Stay updated, free articles. Join our Telegram channel
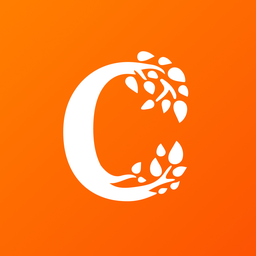
Full access? Get Clinical Tree
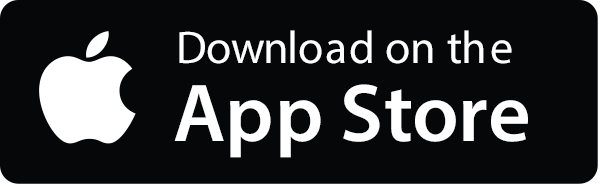
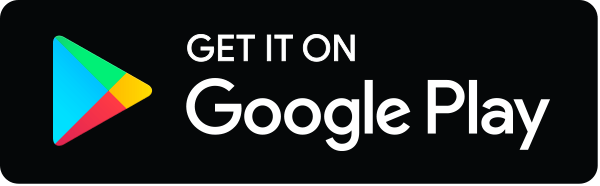