FIGURE 11-1. Schematic representation of the central mechanisms involved in the control of temporal variations in pituitary hormone secretions over the 24-hour cycle. ACTH, Adrenocorticotropic hormone; ANS, autonomous nervous system; FSH, follicle-stimulating hormone; GH, growth hormone; LH, luteinizing hormone; PRL, prolactin; SCN, suprachiasmatic nucleus of the hypothalamus; TSH, thyrotropin.
The first section of this chapter provides an overview of current concepts and recent advances in our understanding of circadian rhythmicity and sleep-wake regulation, and introduces the general properties, physiologic significance, and medical implications of ultradian rhythmicity. This section concludes with a review of the impact of age on these mechanisms. Methodologic aspects specific to the study of hormonal rhythms in human subjects are described in the second section. The third section summarizes the present state of knowledge on circadian and ultradian endocrine rhythms in health and disease for the major endocrine axes. Conditions of altered or abnormal circadian and/or sleep regulation that have implications for the temporal organization of hormonal release are presented in the last section.
Because of limitations on length and in the scope of this chapter, this review is limited to findings in adults. A detailed presentation on the physiology and clinical applications of melatonin serves as the topic of a separate chapter in this volume.
CIRCADIAN RHYTHMICITY
General Characteristics
One of the most obvious characteristics of life on earth is the ability of almost all species to change their behavior on a daily or 24-hour basis. A remarkable feature of these daily or diurnal rhythms is that they are not simply a response to 24-hour changes in the physical environment imposed by the principles of celestial mechanics, but instead arise from an internal time-keeping system1,3 that has the intrinsic capability to continuously generate rhythmic activity over a nearly 24-hour period. Thus, under laboratory conditions devoid of any external time-giving cues, it has been found that nearly all 24-hour rhythms continue to be expressed. However, under such constant conditions, the period of the rhythm rarely remains exactly 24 hours but instead is “about” 24 hours, and this is why these rhythms are referred to as circadian, from the Latin “circa diem,” meaning “around a day.” When a circadian rhythm is expressed in the absence of any 24 hour signals in the external environment, it is said to be free-running. Under free-running conditions, the endogenous period generally is close to, but is nearly never exactly, 24 hours. Strictly speaking, a diurnal rhythm should not be referred to as circadian until it has been demonstrated that such a rhythm persists under constant environmental conditions. The purpose of this distinction is to separate out those rhythms that are simply a response to 24-hour changes in the environment from those that are endogenous. However, for practical purposes, there is little reason to make a distinction between diurnal and circadian rhythms, since an endogenous timing device underlies the generation of almost all diurnal rhythms. In this chapter, we therefore will extend the use of the term circadian rhythm to include all diurnal variations that recur regularly at a time interval of approximately 24 hours.
An immense variety of circadian rhythms have been observed in man. Human circadian rhythms have been characterized for blood constituents such as white blood cells, amino acids, and hormones, innumerable physiologic variables such as body temperature, heart rate, blood pressure, and urinary volume, and behavioral parameters such as food intake, sleep, mood, vigilance, and cognitive performance. Rhythms have also been noted in response to various challenges such as drugs and stress. Circadian rhythmicity is maintained when subjects are sleep deprived, when they are starved, or when they receive equal amounts of food at short intervals over the day. The timing of single meals, however, can have effects on the pattern of at least some variables, including hormones, and the timing, duration, and quality of sleep and wake can alter the expression of many rhythms, especially those of the endocrine system.
The endogenous nature of human circadian rhythms has been established by experiments in which subjects were isolated with no access to the natural light-dark cycle and no time cues. Such experiments were first performed in natural caves, then in underground bunkers, and finally in specially designed windowless soundproof apartments. The results of one such early experiment, conducted in an artificial underground unit in Germany, are shown in Figure 11-2.4 The rest-activity cycle of the subject is plotted horizontally, day by day, and the times of occurrence of the daily maximum of the body temperature cycle are indicated by closed triangles. During the first 7 days of the experiment, the door of the isolation unit was left open, and the subjects knew the time of day. The average duration (t) of the rest-activity cycle and of the rhythm of body temperature was 24 hours. When, thereafter, the subject lived in complete isolation, both rhythms free-ran but with a mean period of about 26 hours. The free-running period varies from one individual to another. In humans, free-running periods of around 25 hours have been observed under conditions of prolonged temporal isolation. However, assessments of the human free-running period based on the so-called forced desynchrony protocol have provided estimations of between 24.1 and 24.2 hours.5
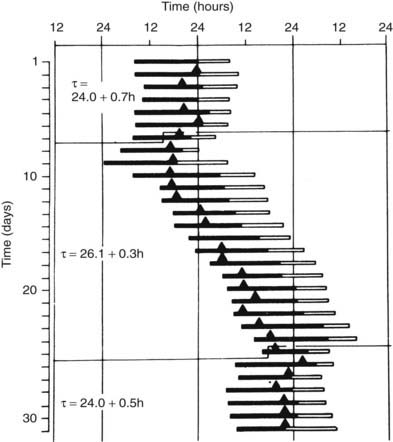
FIGURE 11-2. Circadian rhythms of wakefulness (black bars), sleep (white bars), and maximal rectal temperature (triangles) in a human subject who was exposed to external synchronizing agents for the first and last 7 days and was isolated from all time cues in an underground bunker between days 8 and 24.
The Suprachiasmatic Nucleus: A Master Circadian Pacemaker
In mammals, the suprachiasmatic nucleus (SCN), which consists of two small, bilaterally paired nuclei in the anterior hypothalamus, each of which contains about 10,000 cells in rodents, functions as the master circadian clock. Under both free-running and entrained conditions, destruction of the SCN in a variety of species leads to abolishment or severe disruption of many endocrine, behavioral, and physiologic rhythms.1,6 The role of the SCN as the control center for the circadian system, first suggested by lesion studies, was confirmed by studies involving transplantation of the SCN from one animal to another. Indeed, circadian rhythmicity can be restored in adult arrhythmic SCN-lesioned rodents by transplanting fetal SCN tissue into the region of the SCN.7,8 A number of SCN rhythms, including those of neural firing, vasopressin release, glucose metabolism, and gene expression, persist in vitro.9–12 The ability of SCN cells to generate a circadian signal does not rely on some inherent network property of many cells acting together: Single SCN cells in culture can generate circadian neural signals.13 Neurons within the SCN appear to be organized into two groups: a core group of light responsive but nonrhythmic cells, and a shell of rhythmic cells.14,15
The generation and maintenance of circadian oscillations in the SCN involve a series of clock genes (including per1, per 2, per3, cry1, cry2, tim, clock, B-mal1, and CKIε/δ), often referred to as canonical, which interact in a complex feedback loop of transcription/translation.16,17 Indeed, improved tissue culture techniques and real-time monitoring of the expression of circadian clock genes have revealed that circadian oscillations in gene expression can persist in the SCN in vitro (as well as in other tissues, see later) for many cycles.16 It is important to note that mutations or deletions of canonical circadian clock genes have been found to profoundly affect endocrine rhythms and normal endocrine function in a variety of central and peripheral tissues.18–20 For reviews on the molecular and genetic control of circadian rhythmicity, the reader is referred to references 16, 17, and .
In recent years, it has been recognized that circadian oscillations can be generated in areas of the brain other than the SCN, as well as in many peripheral tissues.9,22 These local oscillators appear to be under the control of the SCN and of the canonical circadian clock genes in the SCN, through the synchronization or entrainment of the same circadian clock machinery at the local tissue level. The SCN controls or synchronizes these autonomous circadian clocks in non-SCN tissues directly via neural and/or endocrine signals, or indirectly via its control of behavioral rhythms such as the sleep-wake cycle and the rhythm of feeding. However, the precise mechanisms are not well understood.
Photic Entrainment of Circadian Rhythms
The fact that the endogenous circadian period is not exactly equal to 24 hours implies that changes in the physical environment must synchronize or entrain the internal clock. Otherwise, a clock with a period only a few minutes shorter or longer than 24 hours soon would be totally out of synchrony with the environmental day. Agents that are capable of entraining or synchronizing circadian rhythms are often called zeitgebers, a German neologism meaning “time giver.”
The light-dark (LD) cycle is the primary agent that synchronizes most circadian rhythms. Thus, in the presence of a 24-hour LD cycle, the period of circadian rhythms exactly matches the period of the LD cycle. In addition to establishing period control, an entraining LD cycle establishes phase control, such that specific phases of the circadian rhythm occur at the same time in each cycle. Entrainment is restricted to cycles with periods that are close to 24 hours in duration and, in general, is not possible for LD cycles that are more than a few hours shorter or longer than the endogenous circadian period. If the period of the LD cycle is too short or too long for entrainment to occur, the circadian rhythm free-runs. This rigidity of the circadian pacemaker has been used in so-called forced desynchrony studies, where subjects are maintained on a light-dark and sleep-wake cycle with a period outside the range of entrainment, such as 20 hours or 28 hours.5,23 As mentioned earlier, such protocols have provided estimations of the endogenous period of the human circadian system that are closer to 24 hours (i.e., averaging 24.1 hours) than those obtained in prolonged studies of temporal isolation.5 The fact that the human endogenous circadian period probably is very close to 24 hours is consistent with the findings of a study showing that a schedule of sleep-wake and dark-light cycles with very low light intensity during wakefulness is able to maintain entrainment to the 24-hour day but not to a 23.5- or 24.6-hour day.24
The eyes are involved in relaying entraining information from the LD cycle to the circadian timing system in mammals via a unique pathway, separate from the visual system, and referred to as the retinohypothalamic tract.25 At the level of the optic chiasm, retinal projections first enter the brain in the region of the SCN and surrounding hypothalamic areas.25 Thus, the integrity of the primary visual centers of the brain and/or of the perception of light is not necessary for entrainment of circadian rhythms by the LD cycle. The independence of photic input to the circadian clock from the visual system in mammals actually is not surprising from an evolutionary point of view. In all nonmammalian vertebrates, entrainment of circadian rhythms can occur in the absence of the eyes and relies on nonretinal photoreceptors in the brain.26 The independence of the circadian light sensing system from the visual system in mammals was suggested in early studies showing that rod-less/cone-less mice could still entrain to the light-dark cycle, even though the eyes were necessary.27 Early in this century, several laboratories discovered almost simultaneously that a special subset of retinal ganglion cells containing melanopsin could act as photoreceptors for relaying light information to the circadian clock in the SCN.28–31 This breakthrough not only demonstrated a new light sensing system in the retina, but also opened up new avenues of research regarding the impact of light on brain function independent of the visual system, including possible neuroendocrine effects.32,33 The finding that visual light perception is not necessary for circadian light perception can explain why in some totally blind humans, light exposure is capable of suppressing melatonin levels, indicating that visual blindness should not be equated with circadian blindness.34 In addition to the retinohypothalamic tract, the SCN receives retinal information indirectly from the lateral geniculate nucleus (LGN), which receives a direct projection from the retina.25
To examine how a zeitgeber such as light influences the circadian system, the organism is maintained in constant conditions (e.g., constant darkness) and then is exposed to the zeitgeber for a brief period (e.g., 1 hour) before it is returned to constant conditions.35 The effects of zeitgeber exposure on a phase reference point of an overt circadian rhythm (e.g., onset of locomotor activity, minimum of body temperature) in subsequent cycles then are determined. A plot of the direction and magnitude of the phase-shift as a function of the circadian time of zeitgeber exposure is called a phase response curve (PRC).36 In the human, light exposure during the late evening and the first half of the usual sleep period results in phase delays, but light exposure at the end of the usual sleep period and in the early morning results in phase advances. The transition from phase delays to phase advances occurs around the time of the minimum of body temperature, that is, at between 04.00 and 06.00 hours in most subjects. The magnitude of the phase-shifts is also wavelength dependent, with exposure to short-wavelength monochromatic light (shifted to the blue) resulting in much larger shifts than exposure to longer-wavelength light of equal photon density,37 because the retinal ganglion cells that contain melanopsin are most sensitive to blue light.
Although differences in amplitude may exist, the general shape and characteristics of the PRC to light pulses are similar for all species. Based on this PRC, appropriate exposure to bright light can accelerate adaptation to shifts such as those occurring in jet lag and shift work. The amplitude of the phase-shifts depends on light intensity and duration, and on the number of consecutive exposures.38 For example, exposure to single 3- to 7-hour light pulses can induce phase-shifts on the order of 1 to 3 hours39–41; repeated exposure for 2 to 3 consecutive days may cause much larger 6- to 12-hour shifts.5,42 Intervening periods of dark/sleep exposure could play a role in enhancing the phase-shifting effects of repeated exposure to light.
Exposure to dark also can cause a phase-shift of mammalian circadian rhythms. Because under most circumstances exposure to dark is associated with changes in activity levels (i.e., increases in activity in nocturnal animals and decreases in activity and/or sleep induction in diurnal animals), it has been unclear whether the phase-shifts occurred in response to dark exposure per se, or in response to associated changes in activity levels.43 A study in hamsters, however, has demonstrated that resetting of the rhythm of locomotor activity and concomitant downregulation of circadian clock gene expression following exposure to dark pulses could occur independently of wheel running activity.44 In humans, abrupt 8-hour advances or 8-hour delays of the sleep-wake cycle result in immediate 2-hour phase-shifts in the same direction.45,46 Daytime naps of 6 hours’ duration in total darkness presented over a background of very dim light were found to cause delay shifts when initiated in the morning and advance shifts when initiated in the evening.47 Naps initiated in the afternoon cause no significant phase-shifts.
Nonphotic Zeitgebers
Nonphotic cues, for example, social and/or behavioral cues, also may alter the rest-activity cycle by eliciting activity during the normal rest period or by preventing activity during the normal active period, resulting in phase-shifts in circadian rhythms of activity, as well as other behavioral, physiologic, and endocrine markers.48–51 In nocturnal rodents, the PRC to activity-inducing stimuli is about 12 hours out of phase with the PRC to light pulses.52
How nonphotic information reaches the SCN still is not known, although evidence from lesion studies suggests that a distinct subdivision of the LGN, the intergeniculate leaflet (IGL), may be involved in mediating the effects of activity on the clock.53 Furthermore, the IGL is the source of neuropeptide Y (NPY) innervation of the SCN, and the administration of NPY into the SCN area, as well as electrical stimulation of the geniculohypothalamic tract, induces phase-shifts in hamster locomotor activity rhythm that are similar to those induced by activity-inducing stimuli.53 The LGN/IGL may be a common pathway by which information about the lighting environment and the activity-rest state reaches the circadian clock, and it may be involved in integrating information from the external and the internal environment. Both the LGN/IGL and the SCN receive a dense serotonergic projection from the midbrain raphe nuclei, and substantial evidence now indicates that these projections play a role in both photic and nonphotic regulation of the mammalian circadian clock.54–56
Nonphotic stimuli may affect human circadian rhythms. Exposure to a single session of 3 hours of moderate-intensity exercise during the usual nighttime period was found to result in phase-shifts of markers of circadian phase on the next day, with the direction and magnitude of the phase-shifts being dependent on the timing of exercise.57 Similar findings were obtained with nocturnal exposure to high-intensity 1 hour exercise sessions.58 Supporting evidence for a zeitgeber effect of exercise was obtained in a field study, which found that adaptation to night work could be facilitated by nocturnal exercise.59 These findings were confirmed by a study demonstrating that daily exposure to nightttime exercise facilitated phase delays in circadian melatonin rhythm, even when subjects were maintained in very dim light.60 Nocturnal exercise of low intensity also causes phase-delays in circadian rhythms in older adults, suggesting that it could be a useful treatment for the adjustment of circadian rhythmicity in older populations.61
Another nonphotic agent that has been shown to induce phase-shifts in human circadian rhythms is melatonin.62 This action of melatonin is often referred to as chronobiotic and is discussed in detail in Chapter 22. Specific neural connections are present between the SCN and the pineal gland, and diurnal variation in plasma melatonin levels is driven by the circadian clock.63 Phase-shifts of the central circadian signal induced by changes in the LD cycle will be faithfully reflected in the synchronization of the onset of nocturnal melatonin secretion.40,64,65 Evidence suggests that, in turn, the melatonin rhythm feeds back on the clock (where melatonin receptors have been identified) and exerts synchronizing effects.63
The timing of feeding, when restricted to a narrow temporal window, can affect the entrainment pattern of behavioral rhythms through what has been referred to as a food-entrainable oscillator that is independent of the SCN. Recent findings in rodents have led to a renewed interest in the role of feeding in overall circadian organization in mammals, including the following: (1) alterations in circadian clock genes can lead to obesity and other metabolic abnormalities,2,20 (2) metabolic transcription factor and nuclear receptors involved in metabolism can alter the expression of circadian clock genes,66,67 (3) the timing of feeding can alter rhythmicity in several peripheral circadian oscillators,20,68 and (4) a high-fat diet can alter behavioral and molecular circadian rhythms in central and peripheral tissues involved in the regulation of energy balance.69 Although controversy exists as to the location of an SCN-independent food entrainable oscillator,70,71 now overwhelming evidence suggesting that the circadian and metabolic systems are linked together at molecular, cellular, and behavioral levels has fueled great interest in the possible role of circadian disorganization in obesity, diabetes, and other cardiometabolic disorders.20,72,73
Sleep-Wake Regulation
General Characteristics
The sleep-wake cycle may be viewed as a 24-hour rhythm driven partially by the circadian pacemaker and partially by the homeostatic regulation of sleep pressure. Sleep itself is an ultradian rhythm in that it involves two states of distinct brain activity, each of which is generated in specific brain regions. The ultradian rhythm of normal sleep is an approximate 90-minute oscillation between non-REM (rapid eye movement) and REM stages. In healthy subjects, this pattern usually is repeated four to six times per night. REM sleep and non-REM sleep are characterized by distinct patterns of cerebral and peripheral activity.
In the normal sequence, sleep is intiated by the lighter stages of non-REM sleep (i.e., stages I and II), followed within 10 to 20 minutes by slow-wave sleep (SWS; stages III and IV). These deeper stages of sleep are maintained for nearly 60 minutes in normal young subjects but usually are much shorter (5 to 10 minutes), if present at all, in older adults. Then, lighter stages of non-REM sleep reappear, and the first REM period is initiated. As the night progresses, non-REM sleep becomes shallower, the duration of REM episodes becomes longer, and the number and duration of awakenings increase. In normal young subjects, approximately 50% of a normal night is spent in stages I and II sleep, 20% in SWS, 25% in REM, and 5% in wake. In adults over 60 years of age, SWS usually is reduced to only 5% to 10% and REM sleep to 10% to 15%, while the proportion of time awake may reach 30% of the night.
During deep non-REM sleep (SWS), the electroencephalogram (EEG) is synchronized with low-frequency, high-amplitude waveforms, referred to as slow waves or delta waves. During REM sleep, eye movements are present, muscle tone is inhibited, and the EEG resembles that of active waking. During REM sleep, cerebral glucose utilization is similar to that of waking, but it is decreased during SWS.
The all-night recording of EEG, muscle tone, and eye movements, called the polysomnogram, is scored visually over 20 or 30 second periods in stages I, II, III, IV, REM, and Wake with the use of standardized criteria.74 This procedure allows determination of the duration of each sleep stage but does not quantify the intensity of non-REM sleep. In contrast, the quantification of EEG recordings by power spectral analysis provides useful information regarding sleep depth or sleep intensity, because spectral analysis is sensitive to the amplitude of the delta waves. Higher-amplitude delta waves reflect more intense, deeper sleep, with less sensitivity to arousal stimuli. Slow-wave activity (SWA), i.e., spectral EEG power in the low-frequency range (also called delta range; 0.5 to 4.0 Hz), is a marker of the intensity of non-REM sleep.
As detailed below, the timing, duration, and architecture of sleep are under the dual control of a homeostatic mechanism that relates sleep pressure to the duration of prior wakefulness and of central circadian rhythmicity.
Neuroanatomic Basis of Sleep Regulation
Normal waking is associated with neuronal activity in regions of the so-called ascending arousal system, which includes monoaminergic neurons in the brain stem and posterior hypothalamus, cholinergic neurons in the brain stem and basal forebrain, and orexin (hypocretin) neurons in the lateral hypothalamus.75,76 Initiation of sleep therefore requires the inhibition of these multiple arousal systems. In recent years, the ventrolateral preoptic area (VLPO) of the hypothalamus has been identified as involved in the inhibition of arousal. The VLPO contains sleep-active neurons that use the inhibitory neurotransmitter GABA (gamma-aminobutyric acid) and have much higher firing rates during deep sleep than during wakefulness.77–79 Lesions of the central cell cluster of the VLPO drastically reduce SW activity. Neurons of the VLPO provide GABAergic inhibitory innervation of the major monoamine arousal systems in the brain stem. Reciprocally, inhibitory pathways result from the monoamine arousal nuclei to the VLPO.80 The orexin neurons in the lateral hypothalamus project to all components of the ascending arousal system and stimulate the cortex.81 REM sleep is regulated primarily by cholinergic nuclei in the pons.
Interactions Between Circadian Rhythmicity and Sleep-Wake Homeostasis
Several features of the interaction between sleep and circadian rhythmicity appear to be fairly unique to the human species. First, human sleep generally is consolidated in a single 6- to 9-hour period, whereas fragmentation of the sleep period in several bouts is the rule in most other mammals. Possibly as a result of this consolidation of the sleep period, the wake-sleep transition in man is associated with physiologic changes that usually are more marked than those observed in animals. For example, the secretion of growth hormone (GH) in normal adults is tightly associated with the beginning of the sleep period, whereas the relationship between GH secretory pulses and sleep stages is much less evident in rodents, primates, and dogs. Second, man is unique in his capacity to ignore circadian signals and to maintain wakefulness despite increased pressure to go to sleep. Finally, approximately 25% of human subjects maintained for prolonged periods in temporal isolation have shown behavioral modifications that have not been observed in laboratory animals under constant conditions. These modifications consist of a desynchronization between the sleep-wake cycle and other rhythms, such as those of body temperature and cortisol secretion, which continue to free-run with a circadian period. Under conditions of so-called internal desynchronization, the sleep-wake cycle may be lengthened suddenly to 30 hours or more, while the rhythm of body temperature continues to free-run with a circadian period.4 Wakefulness may last longer than 30 hours. Remarkably, the subjects are not aware of these drastic changes in their way of living. Instead, most of them believe that they are living on a more or less regular 24-hour schedule. This can be explained by the observation that time perception is altered profoundly: Subjective estimations of 1 hour intervals are positively correlated with the duration of wakefulness.82 Of particular interest is that subjects continue to have three meals per “day,” irrespective of the actual number of hours they are awake.83 The intervals between meals as well as those between wake-up and breakfast, or between dinner and bedtime, are stretched or compressed in strong proportionality to the duration of wakefulness.84 The mechanisms that cause spontaneous internal desynchronization are not completely understood.
Detailed analyses of data obtained during temporal isolation and forced desynchrony protocols show that the timing, duration, and architecture of sleep are regulated in part by circadian rhythmicity.23,85 Thus, the duration of sleep episodes is correlated with the phase of the circadian rhythm of body temperature and not with the duration of prior wakefulness. Short (i.e., 7 to 8 hours) sleep episodes occur in free-running conditions when the subject goes to sleep around the minimum of body temperature, whereas long (i.e., 12 to 14 hours) sleep episodes occur when sleep starts at around the maximum of body temperature. Moreover, the distribution of REM sleep is markedly modulated by circadian timing. In contrast, the hourglass-like mechanism of sleep-wake homeostasis originally was thought to be largely independent of the circadian system and to involve one or several putative neural sleep factors, which rise during waking and decay exponentially during sleep.86 This homeostatic mechanism regulates the timing, amount, and intensity of SWS and SWA. The VLPO has been proposed as a neuroanatomic locus for the interaction of the homeostatic process and central circadian rhythmicity, as it receives dense projections from the dorsomedial hypothalamic nucleus, which itself receives direct and indirect projections from the SCN.87 Based on human studies described later, it is thought that the SCN generates a waking signal that promotes alertness during the active period. In support of this theory, studies have shown that rodents and monkeys with SCN lesions have increased sleep duration.88,89 Furthermore, studies in rats have described an indirect neuronal circuit from the SCN to the locus coeruleus, an area of the midbrain involved in the control of arousal.90 A role for the SCN in promoting sleep at other circadian times is suggested by the finding of decreased sleep in a mouse with a mutation of the Clock gene.91 The recent finding that the mutation or deletion of a number of circadian clock genes affects not only the timing of sleep but also many other sleep-wake traits, including traits linked to the homeostatic drive to sleep,15,91–94 indicates that circadian and homeostatic processes underlying the regulation of the sleep-wake cycle may be linked at molecular and anatomic levels of organization.
The dual control of sleep by circadian and homeostatic mechanisms extends to the control of objective and subjective measures of sleep tendency, mood, and vigilance.95–98 When wakefulness is extended beyond the usual 16 to 18 hours, maximum subjective sleepiness coincides with the minimum of body temperature, mood, and performance. Remarkably, despite continued sleep deprivation, subjective fatigue then decreases, and mood and performance partially recover during the daytime hours, reflecting an interaction of circadian timing with the accumulation of waking time.96–102 Currently, it is believed that the circadian clock generates a waking signal that increases from morning to evening and is expressed maximally in the early evening hours, 1 to 2 hours before the onset of nocturnal melatonin secretion.97 This circadian waking signal counteracts the buildup of the putative factor “S” underlying the homeostatic process, allowing the individual to maintain a high level of alertness throughout the usual waking period. Current data from human studies are compatible with the hypothesis that the SCN generates a sleep signal in the early evening hours.103
Circadian rhythmicity and sleep-wake homeostasis also interact to regulate hormonal secretion. These modulatory effects were long thought to be present only in hormones directly dependent on the hypothalamic-pituitary axis. However, it is now clear that modulation by circadian rhythmicity and sleep is also present in other endocrine systems, such as glucose regulation and the renin-angiotensin system.104,105 The multiple pathways by which circadian rhythmicity, sleep-wake homeostasis, and their interaction modulate hormonal release are incompletely understood. As is illustrated in Fig. 11-1, humoral and/or neural signals originating from the hypothalamic circadian pacemaker and from brain regions involved in sleep regulation affect the pulsatile release of hypothalamic neuroendocrine factors, which stimulate or inhibit intermittent secretion of pituitary hormones. The autonomic nervous system is another pathway linking the central control of sleep-wake homeostasis and circadian rhythmicity with peripheral endocrine organs. It appears that stimulatory or inhibitory effects of sleep on endocrine release are primarily associated with SW rather than REM sleep.106–110 Pituitary hormones that influence endocrine systems not directly controlled by hypothalamic factors probably mediate, together with the autonomous nervous system, the modulatory effects of sleep and circadian rhythmicity on these systems (e.g., counterregulatory effects of GH and cortisol on glucose regulation105).
To delineate the relative roles of circadian and sleep effects in the temporal organization of hormonal secretion, strategies based on the fact that circadian rhythmicity needs several days to adapt to abrupt shifts in the sleep-wake cycle have been used. Thus, by shifting sleep times by 8 to 12 hours, masking effects of sleep on circadian inputs are removed, and the effects of sleep at an abnormal circadian time are revealed. Fig. 11-3 illustrates the mean profiles of plasma cortisol, GH, prolactin, and thyrotropin (TSH) as observed in normal subjects who were studied before and during an abrupt 12-hour shift in the sleep-wake and dark-light cycles. The study period extended over a 53-hour span and included an 8-hour period of nocturnal sleep, a 28-hour period of continuous wakefulness, and a daytime period of recovery sleep. To eliminate the effects of feeding, fasting, and postural changes, subjects remained recumbent throughout the study, and the normal meal schedule was replaced by intravenous glucose infusion at a constant rate. As shown in Figure 11-3, this drastic manipulation of sleep had only modest effects on the wave shape of the cortisol profile, in sharp contrast with the immediate shift of GH and prolactin rhythms, which followed the shift in the sleep-wake cycle. As will be reviewed in subsequent sections, numerous studies have indicated that control of diurnal rhythms of corticotropic activity is primarily dependent on circadian timing, whereas sleep-wake homeostasis appears to be an important factor in control of the 24-hour profiles of GH and prolactin.111 Nevertheless, small modulatory effects of sleep-wake homeostasis on cortisol secretion and, conversely, influences of circadian timing on somatotropic function have been clearly demonstrated.112 The diurnal variation in TSH levels includes an evening elevation that is thought to be under circadian control and nocturnal inhibition by sleep-dependent processes that is clearly demonstrated during sleep deprivation, when a large increase in nocturnal TSH levels is apparent, as is shown in the lower panel of Fig. 11-3.111
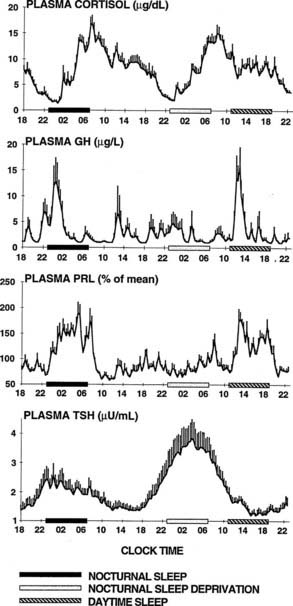
FIGURE 11-3. Mean (+standard error of the mean [SEM]) 24-hour profiles of plasma cortisol, growth hormone (GH), prolactin (PRL), and thyrotropin (TSH) in a group of eight normal young men (20 to 27 years old) studied during a 53-hour period that include 8 hours of nocturnal sleep, 28 hours of sleep deprivation, and 8 hours of daytime sleep. The black bars represent the sleep periods. The open bars represent the period of nocturnal sleep deprivation. The dashed bars represent the period of daytime sleep. Data were sampled at 20-minute intervals.
(From Van Cauter E, Spiegel K: Circadian and sleep control of endocrine secretions. In Turek FW, Zee PC [eds]: Neurobiology of Sleep and Circadian Rhythms, vol 133. New York, Marcel Dekker, 1999, pp 397–426.)
Hormonal profiles thus are easily measurable reflections of central mechanisms of biologic time keeping. In clinical investigations of conditions of abnormal circadian rhythmicity such as jet lag, and in human studies of the effects of exposure to natural or artificial zeitgebers, hormonal profiles are commonly used as markers of the status of the circadian clock and of its interactions with sleep.
ULTRADIAN RHYTHMICITY
Range of Ultradian Rhythms
The term ultradian is used primarily to designate rhythms with periods ranging from fractions of hours to several hours. Ultradian oscillations often are less regular and less reproducible than circadian rhythms. In most cases, they appear to represent an optimal functional status within the system where they occur rather than serving the primary function of a clock, that is, an accurate time measuring device. A wide variety of ultradian rhythms have been noted. The most prominent include pulsatile hormonal release and the alternation of REM and non-REM stages in sleep. In the human, the approximately 90-minute REM–non-REM cycle is accompanied by similar periodicity of dreaming, penile erections, sympathovagal balance, and breathing. It has been suggested that this ultradian rhythm during sleep may be a reflection of a basic rest-activity cycle (BRAC), which would occur during wakefulness as well.113 This concept has received some experimental support from a study demonstrating the existence of an ultradian rhythm of brain electrical activity in the frequency range of 13 to 35 Hz, an index of central alertness, during waking.114 It is interesting to note that pulses of cortisol release were significantly associated with ultradian oscillations in alertness.114
Oscillations at frequencies higher than the hourly (i.e., circhoral) range characterizing pulsatile release have been observed for a variety of hormones and metabolic variables. In particular, rapid oscillations of insulin secretion with periods in the 10- to 15-minute range have been well characterized in humans.115,116 Oscillations with a similar period also characterize lipolysis from omental fat, resulting in pulsatile free fatty acid release that appears driven by bursts of sympathetic drive to the adipocytes.117
Properties and Clinical Implications of Pulsatile Hormonal Release
In the endocrine system, ultradian variations have been observed for anterior and posterior pituitary hormones, for hormones under direct pituitary control, and for other endocrine variables such as parathyroid hormone, norepinephrine, plasma renin activity, and leptin and insulin secretion. The interval of recurrence of pulses varies from hormone to hormone and from species to species. The relative importance of pulsatile or oscillatory secretory activity versus tonic release also varies from one axis to another. For some hormones, secretory activity appears to be entirely pulsatile, with no detectable secretion between pulses. In normal men, evidence suggestive of intermittent secretion without tonic release has been obtained for luteinizing hormone (LH), follicle-stimulating hormone (FSH), GH, and adrenocorticotropic hormone (ACTH).118–120 For some hormones, pulsatile release is superimposed on a tonic level of secretion, or secretion occurs continuously but is increased and decreased in an oscillatory fashion. Pancreatic insulin secretion is a well-established example of this type of ultradian oscillation.121,122 Evidence for the existence of tonic secretion also has been obtained for pituitary prolactin and TSH release.118,119
The pulsatile nature of hormonal release implies that changes well in excess of 100% may occur within 1 hour. Therefore, it is necessary to obtain multiple samples to estimate the mean circulating level of many hormones and to determine the presence or absence of a circadian rhythm.
The physiologic significance of pulsatile hormone secretion was first proved when the essential role of the episodic nature of gonadotropin-releasing hormone (GnRH) release for normal functioning of the pituitary-ovarian axis was demonstrated.123 Landmark studies showed that continuous infusions of exogenous GnRH in Rhesus monkeys with lesions of the arcuate nucleus, which abolished endogenous GnRH production, inhibited the secretion of LH and FSH. In contrast, the pulsatile administration of the synthetic hypothalamic hormone at a rate of one 6-minute pulse per hour restored normal LH and FSH levels.123 Furthermore, if the rate of pulse delivery was increased to three pulses per hour, or if it was decreased to one pulse every 2 hours, serum LH and FSH levels were partially inhibited. These findings were rapidly applied to treatment for a variety of disorders of the pituitary-gonadal axis124 and led the way to the discovery of the functional significance of pulsatility in other endocrine systems. For example, it was found that oscillatory administration of insulin with a period matching that of the normal pulsatility of endogenous insulin secretion is more effective in lowering glucose levels than is constant infusion.125
IMPACT OF AGING ON MECHANISMS CONTROLLING ENDOCRINE RHYTHMS
Age-related changes in endocrine, metabolic, and behavioral circadian rhythms have been reported in a variety of species, including humans.126–128 One of the most prominent changes is a reduction in rhythm amplitude. The overall findings of a study that examined age-related differences in 24-hour endocrine rhythms in healthy subjects are shown in Fig. 11-4.126 A marked decrease in nocturnal release of TSH, melatonin, prolactin, GH, and melatonin was observed among older volunteers. The amplitude of the cortisol rhythm was decreased among elderly men, primarily because of an elevation of the nocturnal nadir. A retrospective analysis of polygraphic sleep recordings and concomitant profiles of plasma GH and cortisol from 149 normal healthy men, ages 16 to 83 years, showed a different rate of aging of SW sleep and REM sleep129 (Fig. 11-5). SW sleep decreased markedly from early adulthood to midlife and was replaced by lighter sleep (stages I and II) without significant increases in sleep fragmentation or decreases in REM sleep. The transition from midlife to late life involved an increase in wake at the expense of both non-REM and REM sleep. The chronology of aging of GH secretion paralleled that of SW sleep. In contrast, the elevation in evening cortisol levels became significant only after 50 years, when sleep became more fragmented and REM sleep declined. These results were confirmed by a meta-analysis of 65 studies, which found that most of the impact of age on sleep occurs before midlife.130 Despite the fact that older women have more subjective sleep complaints than men,131–133 it was generally assumed that SWS and SWA are less affected by aging in women than in men.134 However, when SWA in non-REM sleep is expressed relative to SWA in REM sleep (i.e., accounting for background SWA), SWA is actually lower in older women than in older men, consistent with the higher frequency of subjective complaints.135
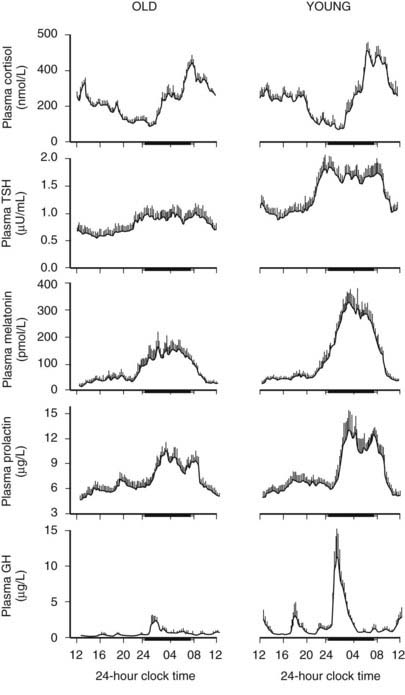
FIGURE 11-4. Mean (+standard error of the mean [SEM]) 24-hour profiles of plasma cortisol, thyrotropin (TSH), melatonin, prolactin (PRL), and growth hormone (GH) levels in eight old (67 to 84 years) and eight young (20 to 27 years) subjects. Data were sampled at 15-minute intervals. The black bars represent the mean sleep period.
(From van Coevorden A, Mockel J, Laurent E, et al: Neuroendocrine rhythms and sleep in aging men. Am J Physiol 260:E651–E661, 1991.)
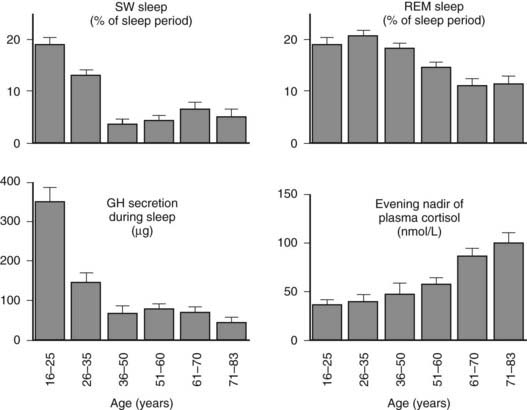
FIGURE 11-5. Left, Slow-wave (SW) sleep and growth hormone (GH) secretion during sleep as a function of age. Note the temporal concomitance between the decrease in SW sleep and in GH secretion. Right, Rapid eye movement (REM) sleep and level of evening nadir of plasma cortisol as a function of age. Note the temporal concomitance between the decrease in REM sleep and the increase in evening cortisol levels. Values shown are means (+standard error of the mean [SEM]) for each age group. Data were obtained in 149 healthy men, ages 16 to 83 years.
(Data from Van Cauter E, Leproult R, Plat L: Age-related changes in slow-wave sleep and relationship with growth hormone and cortisol levels in healthy men. JAMA 284:861–868, 2000.)
Deficits in the maintenance and quality of nocturnal sleep in older adults are paralleled by decreased alertness, attention and memory deficits, and decreased performance during the daytime.136 In both rodents and humans, many circadian rhythms are advanced under entrained conditions such that specific phase points of these rhythms occur earlier than in young subjects.126,137 Both amplitude reduction and phase advance of the rhythm of body temperature have been observed in elderly subjects, and these alterations in circadian regulation were closely associated with changes in sleep-wake habits (i.e., earlier bedtimes and wake times).127,138
Age-related changes in the amplitude and/or the phase of circadian rhythms could be due to changes in the inner workings of the master clock, to alterations in the input pathways to the clock, or to factors downstream between the circadian clock and the system that is expressing the rhythm. Some early studies139,140 have reported that the free-running period of various rhythms in rodents is systematically shortened with age, suggesting that the circadian clock itself is altered in advanced age. This concept has been confirmed in rats via measurement of Per1-luc expression in transgenic animals.141 Age-related phase advances of a number of different behavioral and endocrine rhythms are consistent with the hypothesis that the period of the human circadian clock is shorter in the elderly. However, a study that measured the free-running period in healthy young and older adults using the forced desynchrony protocol has found no age difference.5 Nevertheless, the very healthy older individuals who participated in this demanding protocol exhibited marked decreases in sleep consolidation and had greater difficulties sleeping at adverse circadian phases than did young subjects. These alterations and the clear advance of the propensity to awaken from sleep are thought to be related to both a reduction in the homeostatic drive to sleep and a reduction in the strength of the circadian signal.142 In older adults, in contrast to young subjects, no significant correlation has been noted between the length of the circadian period and the phase angle of entrainment.143
Studies in rodents indicate that aging is associated with decreased responsiveness to the phase-shifting effects of both photic and nonphotic stimuli. Old hamsters show a decreased response to the phase-shifting effects of low-intensity light pulses.144 This observation raises the possibility that in old age, signal transmission of light information to the SCN itself may be decreased, or that SCN responsiveness to photic stimulation may be lessened. Similarly, although induction of locomotor activity during a time of normal inactivity can induce pronounced phase-shifts in the circadian rhythm of locomotor activity in young animals, in old animals the response is greatly diminished or is completely abolished.145,146 It is interesting to note that transplantation of fetal SCN tissue into the SCN region of old hamsters with an intact SCN can restore the response to the phase-shifting effects of triazolam on the activity rhythm.147
Behavioral changes in the elderly also may lead to changes in environmental inputs to the clock. In older adults, exposure to bright light and social cues, both potential entraining agents, is markedly diminished when compared with that seen in young adults.148–150 Furthermore, age-related lens pigmentation decreases the transmission of blue light (i.e., the wavelength to which the circadian system is most sensitive) to the retina.151 Absence of professional constraints, decreased mobility due to illness, and reduced socialization and outdoor activities are all hallmarks of old age. Thus, decreased exposure to environmental stimuli that entrain circadian rhythms could contribute to disruptions in circadian rhythmicity. The use of exposure to bright light and enriched social schedules to reinforce circadian rhythms in older adults and improve nighttime sleep and daytime alertness has proved beneficial in several studies.152 In elderly insomniacs living in a nursing home with limited exposure to environmental light, supplementary light exposure during the middle of the day significantly increased nocturnal melatonin secretion without circadian phase-shifting.153 Evidence indicates that older adults are capable of phase-shifting to the same extent as younger subjects in response to appropriately timed light exposure.154
The ultradian rhythmicity of pulsatile hormonal release is also affected by aging. For a number of individual hormones, including GH, insulin, LH, and ACTH, analyses of 24-hour profiles have shown increased irregularity, or disorderliness, of pulsatile release. Furthermore, the synchrony of pulsatile release between the pituitary hormone and the peripheral hormone (e.g., LH and testosterone, ACTH and cortisol) is partially disrupted in healthy older adults.155
Methodologic Aspects of the Study of Endocrine Rhythms
EXPERIMENTAL PROTOCOLS
Most investigations of circadian rhythms of hormonal release are based on a transversal design, that is, a group of individuals are studied for a minimum of 24 hours each, following the same experimental protocol. The demonstration of circadian rhythmicity then is based on the observation of consistently reproducible characteristics in the observed set of temporal profiles. The group of subjects should be as homogeneous as possible, not only in terms of physical parameters such as age and sex, but also in terms of living habits such as sleep-wake cycles, exercise habits, and meal schedules. Subjects who have regular social habits and describe themselves as “good sleepers” should be preferred. Shift workers and subjects who have made a transmeridian flight less than 2 months before the experiment should be excluded. Before the experiment is begun, volunteers should be asked to adhere to a standardized schedule of meals and bedtimes for several days so as to maximize interindividual synchronization. The use of continuous wrist activity recording during the prestudy period is a convenient way to monitor compliance. At least 1 night of habituation to the laboratory environment and recording procedures should be included. To avoid disruptions in sleep due to the sampling procedure, the catheter should be connected to tubing that extends to an adjoining room during the night. Because of the modulatory effects exerted by sleep stages on hormonal release, it is important to obtain polygraphic sleep recordings by using standardized methods for recording and scoring. Daytime naps should be avoided. The catheter should be inserted at least 2 hours before the first sample is collected so that observation of the effects of venipuncture stress can be avoided. To obtain valid estimations of the circadian parameters, it is necessary to sample at intervals not exceeding 1 hour.
If hormonal profiles are measured as markers of the output of the central circadian oscillator, direct effects of other factors should be minimized. Sleep-wake transitions, meals, stressful activities, and changes in physical activity all may affect hormonal levels. To eliminate these “masking” effects, experimental protocols usually referred to as constant routines have been developed to reliably derive estimates of circadian amplitude and phase from temporal patterns of peripheral hormones and other physiologic variables, such as body temperature. Constant routine conditions generally involve a regimen of continuous wakefulness, constant recumbent posture, constant illumination, and constant caloric intake either under the form of hourly identical aliquots of liquid diet or solid food, or under the form of a constant glucose infusion. Although such constant routine conditions have been used extensively in basic studies of human circadian rhythmicity, the sleep deprivation inherent to this protocol is an obvious limitation. The use of circadian markers that are not masked by sleep, meals, and other factors, such as the 24-hour profile of melatonin, has been advocated. Finally, if only circadian phase, and not amplitude, is of interest, measurements of saliva levels of melatonin during the evening provide a noninvasive way to observe the timing of the onset of nocturnal melatonin secretion, a neuroendocrine event timed by the circadian clock.
In characterizing episodic hormonal fluctuations, consideration of the total amount of blood withdrawn and of the amount of plasma needed to assay the hormones under study is obviously essential to the definition of an adequate sampling protocol. The definition of an optimal sampling protocol depends thus on the type of phenomenon under study. Sampling rates of 1 and 2 minutes will uncover high-frequency, low-amplitude episodic variations superimposed on the slower pulsatile release at intervals of 1 to 2 hours. Sampling rates of 20 and 30 minutes will detect only major pulses lasting longer than 1 hour. As compared with older studies, ultrasensitive assays now available allow detection of small-amplitude pulses at low hormonal concentrations, resulting in the finding of additional, previously undetected, secretory episodes.
PROCEDURES TO QUANTIFY CIRCADIAN VARIATIONS
Among the methods proposed for and applied to the analysis of 24-hour profiles of blood components, the oldest is the Cosinor test.156 The major disadvantage of this test and of its derivatives is its assumption that the observed profile may be described adequately by a single sinusoidal curve. This assumption is practically never met for biological rhythms that are asymmetric in nature (e.g., the sleep-wake cycle is a 08:16 alternation, not a 12:12). Therefore, the Cosinor test generally provides unreliable estimations of rhythm parameters. Unfortunately, in recent years, the Cosinor analysis has regained popularity and has been applied indiscriminately to asymmetric profiles.
Other procedures for the detection and estimation of circadian variation have been based on periodogram calculations or on nonlinear regression procedures.157,158 These methods provide an adequate description of asymmetric wave shapes. The times of occurrence of the maximum and the minimum of the best-fit curve often are referred to as the acrophase and the nadir, respectively. The amplitude of the rhythm may be estimated as 50% of the difference between the maximum and the minimum of the best-fit curve. With the periodogram procedure, confidence intervals for the amplitude, acrophase, and nadir may be calculated.
PROCEDURES TO QUANTIFY PULSATILE HORMONAL SECRETION
Analysis of pulsatile variations may be considered at two levels.159 One may wish to define and characterize significant variations in peripheral levels, based on estimations of the size of the measurement error (i.e., primarily assay error). However, under certain circumstances, it is possible to mathematically derive secretory rates from the peripheral concentrations.160–162 This procedure, often referred to as deconvolution, often reveals more pulses of secretion than does analysis of peripheral concentrations. It also more accurately defines the temporal limits of each pulse. However, deconvolution involves amplification of measurement error, with increased risk for false-positive error. Whether peripheral concentrations or secretory rates are examined, two major approaches are used to analyze the episodic fluctuations. The first and most commonly used is the time domain analysis, wherein the data are plotted against time, and pulses are detected and identified. The second is the frequency domain analysis, in which amplitude is plotted against frequency or period. The time domain analysis provides an estimation of pulse frequency, calculated as the total number of pulses detected divided by the duration of the study period. The regularity of pulsatile behavior may be quantified by examining the distribution of interpulse intervals. Alternatively, the issue of regularity of pulsatile behavior may be approached by examining the distribution of spectral power in a frequency domain analysis.163 Finally, another analytic tool, the approximate entropy (ApEn), has been introduced to quantify the regularity of oscillatory behavior in endocrine and other physiologic time series.164,165
A number of computer algorithms for identification of pulses of hormonal concentration have been proposed. A detailed presentation of the operating principles of each of these procedures is beyond the scope of this chapter. Review articles166,167 have provided comparisons of performance of several pulse detection algorithms. These comparisons have indicated that ULTRA and CLUSTER perform similarly when used with appropriate choices of parameters.167
Endocrine Rhythms in Health and Disease
Diurnal and/or ultradian oscillations have been observed in essentially all endocrine systems. An exhaustive review of all such observations is not possible. The following summary of findings therefore will be limited to the various hypothalamic-pituitary axes, parathyroid hormone, hydromineral hormones, glucose and insulin, and hormones involved in appetite regulation.
PITUITARY AXES
The Corticotropic Axis
Normal Rhythms of Adrenocorticotropic Hormone and Adrenal Secretions
Outputs from the SCN activate rhythmic release of corticotropin-releasing hormone (CRH) that stimulates circadian ACTH release. The 24-hour rhythm of adrenal secretion is primarily dependent on the diurnal pattern of ACTH release. In addition, neuronal signals generated by the SCN are transmitted by a multisynaptic neural pathway to the adrenal cortex.168 The presence in the adrenal cortex of an intrinsic circadian oscillator consisting of interacting positive and negative feedback loops in circadian gene expression has been demonstrated in various animals, including monkeys,169–171 and it has been shown that this adrenal circadian pacemaker gates the physiologic adrenal response to ACTH (i.e., defines a time window during which the adrenal most effectively responds to ACTH).172
The 24-hour profiles of ACTH and cortisol show an early morning maximum, declining levels throughout daytime, a quiescent period of minimal secretory activity centered around midnight, and an abrupt elevation during late sleep, resulting in an early morning maximum. Mathematical derivations of secretory rates from plasma concentrations have suggested that the 24-hour profile of plasma cortisol reflects a succession of secretory pulses of magnitude modulated by a circadian rhythm with no evidence of tonic secretion.120,173 In normal conditions, the acrophase of the pituitary-adrenal periodicity occurs between 06.00 and 10.00 hours. With a 15-minute sampling interval, 12 to 18 significant pulses of plasma ACTH and cortisol can be detected per 24-hour span.174 Circadian and pulsatile variations parallel to those of cortisol have been demonstrated for the plasma levels of several other adrenal steroids, in particular dehydroepiandrosterone (DHEA).175 The temporal concomitance of 24-hour profiles of ACTH, cortisol, and DHEA is illustrated in Fig. 11-6.
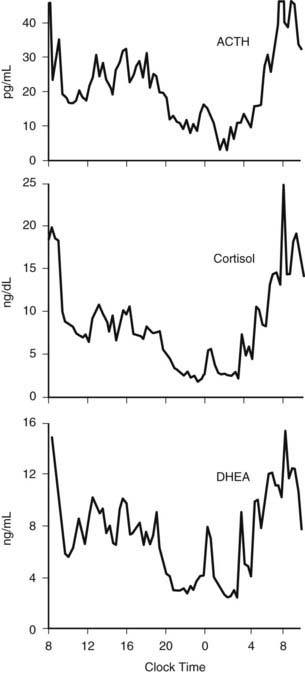
FIGURE 11-6. Twenty-four-hour profiles of plasma adrenocorticotropic hormone (ACTH), cortisol, and dehydroepiandrosterone (DHEA) levels sampled at 20-minute intervals in a healthy young man. Note the temporal concomitance of circadian and pulsatile variations of the three hormones.
(Unpublished data kindly provided by Dr. K. Spiegel.)
The profile shown in the upper panel of Fig. 11-3 illustrates the remarkable persistence of the cortisol and, by inference, ACTH secretory rhythm when sleep is manipulated. Indeed, the overall wave shape of the profile was not markedly affected by the absence of sleep or the presence of sleep at an abnormal time of day. Thus, this rhythm is controlled primarily by the circadian pacemaker.
However, modulatory effects of sleep-wake homeostasis have been clearly demonstrated. As illustrated in Fig. 11-7, sleep onset is consistently associated with short-term inhibition of cortisol secretion, which may not be detectable when sleep is initiated in the morning (i.e., at the peak of corticotropic activity).176–179 This inhibitory effect of sleep appears to be related to SW sleep.109,180 Conversely, final awakenings from sleep, as well as transient awakenings that interrupt the sleep period, consistently trigger pulses of cortisol secretion (see Fig. 11-7),120,178,180–183 and the number of nocturnal microarousals predicts morning plasma and saliva cortisol levels.184 In an analysis of cortisol profiles during nocturnal sleep, it was observed that all transient awakenings that interrupt sleep and last at least 10 minutes were followed within the next 20 minutes by significant bursts of cortisol secretion.183 In addition, a temporal coupling between pulses of cortisol secretion and ultradian variations in an EEG marker of alertness has been reported.114 Total nocturnal wake time is associated with increased 24-hour plasma cortisol concentrations,185 and chronic insomnia with reduced total sleep time is associated with higher cortisol levels across the night.186
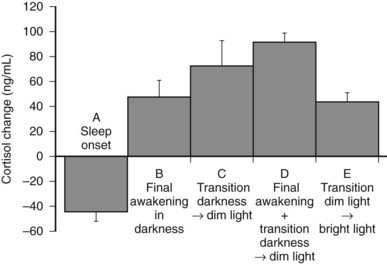
FIGURE 11-7. Mean (and standard error of the mean [SEM]) changes in plasma cortisol levels: A, Within 120 minutes following sleep onset at 15.00 hours (n = 32). B, Within 20 minutes following final spontaneous awakening in darkness (scheduled sleep period 23.00 to 07.00 hours or 15.00 to 23.00 hours; n = 10). C, Within 20 minutes following transition from darkness to dim light at 07.00 or 23.00 hours in subjects awake at bed rest (n = 10). D, Within 20 minutes following final awakening concomitant with transition from darkness to dim light at 07.00 or 23.00 hours (n = 38). E, Within 15 minutes following transition from dim to bright light at 05.00 hours in subjects awake at bed rest (n = 8).
(Panels A to D, Data from Caufriez A, Moreno-Reyes R, Leproult R, et al: Immediate effects of an 8-h advance shift of the rest-activity cycle on 24-h profiles of cortisol. Am J Physiol 282:E1147–E1153, 2002; panel E, Data from Leproult R, Colecchia EF, L’Hermite-Balériaux M, et al: Transition from dim to bright light in the morning induces an immediate elevation of cortisol levels. J Clin Endocrinol Metab 86:151–157, 2001.)
Modulatory effects of dark-light transitions also have been noted. Cortisol secretory pulses associated with morning awakening are enhanced by increasing light intensity.187 Moreover, the transition from darkness to dim light and from dim to bright light may stimulate cortisol secretion in subjects who are awake at bed rest.183,188 The stimulatory effects of bright light exposure on nocturnal cortisol levels appear to be dependent on the timing of exposure.189 When dark-light and sleep-wake transitions occur concomitantly, associated cortisol elevations are nearly two times as high as when the final awakening occurs in continuous darkness183 (see Fig. 11-7). Thus, under usual bedtime schedules, both sleep-wake and dark-light transitions amplify the effects of circadian rhythmicity.
Studies of the 24-hour cortisol profile in the course of adaptation to shifts of the sleep-wake cycle have demonstrated that the end of the quiescent period, which coincides with the onset of the early morning rise, takes longer to adjust and appears to be a robust marker of circadian timing. Twin studies have demonstrated that the timing of the nadir is influenced by genetic factors,190 providing evidence for genetic control of the human circadian phase. In contrast, the timing of the morning acrophase is more labile and may be influenced by the timing of sleep offset,182 the transition from dark to bright light,187 and breakfast intake.191 Finally, anticipation of the expected time of waking has been reported to be associated with a rise in levels of ACTH, but not cortisol, during the end of the sleep period.192
In addition to the immediate modulatory effects of sleep-wake transitions on ACTH and cortisol levels, acute total or partial (4 hours in bed) nocturnal sleep deprivation results in elevated cortisol concentrations in late afternoon and evening on the following day,193 and the amplitude of the cortisol circadian variations is reduced by approximately 15%. Similarly, as illustrated in Fig. 11-8, recurrent partial sleep deprivation (4 hours in bed per night for 6 nights) also results in an elevation of cortisol levels in the late afternoon and evening.194 These disturbances, which were observed in young healthy subjects, are strikingly similar to those noted in older healthy subjects with normal sleep schedules.126,195,196 In any case, sleep loss appears to delay the return to quiescence of the hypothalamic-pituitary-adrenal axis (HPA) that normally occurs in the evening. This suggests that sleep loss, similar to aging, may slow down the rate of recovery of the HPA axis response following a challenge and therefore could facilitate the development of central and peripheral disturbances associated with glucocorticoid excess, in particular when cortisol concentrations are elevated at the time of the normal daily nadir, such as memory deficits, insulin resistance, and osteoporosis.197–201 Conversely, decreased HPA resiliency results in HPA hyperactivity that inhibits SW sleep and promotes nocturnal awakenings, initiating a feed-forward cascade of negative events generated by both HPA and sleep disruptions.
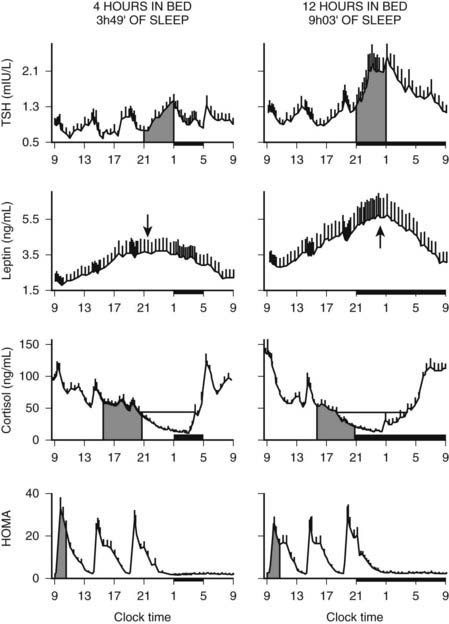
FIGURE 11-8. Impact of recurrent sleep curtailment (4 hours in bed for 6 nights) and of sleep recovery (12 hours in bed for 6 nights) on 24-hour plasma profiles of thyrotropin (TSH), leptin, and cortisol, and on homeostatic model assessment (HOMA) in healthy young men. HOMA was calculated as glucose concentration (mg/dL) × insulin concentration (µU/mL). Values shown are means ± SEM.
(Adapted from Spiegel K, Leproult R, Van Cauter E: Impact of sleep debt on metabolic and endocrine function. Lancet 354:1435–1439, 1999, and from Spiegel K, Leproult R, L’Hermite-Balériaux M, et al: Leptin levels are dependent on sleep duration: relationships with sympathovagal balance, carbohydrate regulation, cortisol and thyrotropin. J Clin Endocrinol Metab 89:5762–5771, 2004.)
The circadian rhythm of cortisol persists throughout adulthood and has been observed through the ninth decade.126,196 Among young adults, 24-hour cortisol levels are slightly lower in women than in men, primarily because of lower morning maxima. With aging, evening cortisol levels increase progressively in both men and women, so that the cortisol nadir is markedly higher in healthy subjects over 70 years of age than in young adults (see Fig. 11-4). It is interesting to note that this elevation of evening cortisol levels occurs with a chronology similar to that observed for a progressive decrease in the duration of REM sleep129 (see Fig. 11-5). As a result, older subjects have elevated 24-hour mean cortisol levels and reduced amplitude of cortisol variations. In addition, the timing of the nadir is advanced by 1 to 2 hours, indicating that aging is associated with an advance in the circadian phase.126,196
In pregnancy, placental CRH is secreted into the maternal circulation in a pulsatile but not in a circadian fashion, and no correlation has been noted between maternal levels of CRH and ACTH. However, ACTH and cortisol concentrations remain strongly correlated with each other over time, suggesting that diurnal variation in maternal ACTH probably is driven by another ACTH secretagogue.202
Alterations in Disease States
The 24-hour profile of pituitary-adrenal secretion remains largely unaltered in a wide variety of pathologic states. Disease states in which pronounced alterations of the cortisol rhythm have been observed include primarily (1) disorders involving abnormalities in binding and/or metabolism of cortisol; (2) the various forms of Cushing’s syndrome; and (3) depression and posttraumatic stress disorder (PTSD).
The relative amplitude of the circadian rhythm and of the episodic fluctuations in cortisol is blunted in patients with liver disease203 and in those with anorexia nervosa,204 primarily because of the decreased metabolic clearance of cortisol. Pulsatile secretion of ACTH, but not cortisol, was reportedly enhanced in obese premenopausal women.205 In hypothyroid patients, the mean level is markedly elevated and the relative amplitude of the rhythm is dampened.206 These alterations are thought to be due to both diminished clearance and decreased efficiency of feedback control. In contrast, in hyperthyroidism, where cortisol production and peripheral metabolism are increased, episodic pulses are enhanced.207
A low-amplitude circadian variation may persist in pituitary-dependent Cushing’s disease. Cortisol pulsatility is blunted in about 70% of patients with Cushing’s disease, suggesting autonomous tonic secretion of ACTH by a pituitary tumor. However, in about 30% of these patients, the magnitude of the pulses is enhanced instead.208 These hyperpulsatile patterns could be caused by enhanced hypothalamic release of CRH or persistent pituitary responsiveness to CRH. It also has been shown that patients with Cushing’s disease secrete ACTH and cortisol jointly more asynchronously than healthy subjects.209 The left and middle panels of Fig. 11-9 compare representative and mean 24-hour cortisol profiles in normal subjects and in patients with Cushing’s disease.
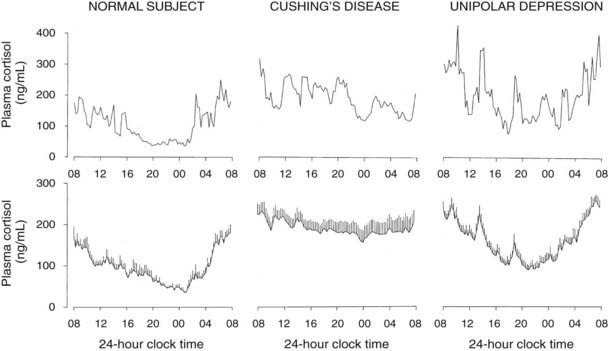
FIGURE 11-9. Twenty-four-hour profiles of plasma cortisol in normal subjects, patients with pituitary Cushing’s disease, and patients with major endogenous depression of the unipolar subtype. For each condition, a representative example is shown in the top panel, and mean (+standard error of the mean [SEM]) profiles from 8 to 10 subjects are shown in the lower panel.
(From Van Cauter E: Physiology and pathology of circadian rhythms. In Edwards CW, Lincoln DW [eds]: Recent Advances in Endocrinology and Metabolism. Edinburgh, Churchill Livingstone, 1989, vol 3, pp 109–134.)
In patients with primary adrenal Cushing’s syndrome, increased cortisol secretion appears to result from both increased basal secretion and increased pulse frequency.210 Although the persistence of a low-amplitude circadian cortisol variation has been claimed,210 examination of the individual profiles does not support this notion. Nevertheless, the partial persistence of cortisol rhythmicity in the absence of ACTH could reflect the newly described neural pathway between the SCN and the adrenal cortex.168
The absence or even the dampening of cortisol circadian variations in Cushing’s syndrome has obvious implications for clinical diagnosis, as time of day when plasma samples are obtained has to be taken into account during evaluation of the result. Differentiation between normal and pathologic levels may be greatly improved by adequate selection of the sampling time, because the overlap between normal individual values and values in patients with Cushing’s syndrome is minimal during a 4-hour interval centered around midnight.
Hypercortisolism with persistent circadian rhythmicity and increased pulsatility is found in a majority of severely depressed patients.211–213 This is illustrated in the right panels of Fig. 11-9. In these patients, who do not develop the clinical signs of Cushing’s syndrome despite high circulating cortisol levels, the quiescent period of cortisol secretion is shorter and more fragmented, and it often starts later and ends earlier than in normal subjects of comparable age. These alterations could reflect the impact of sleep disturbances, as well as an advance in circadian phase. When clinical remission of the depressed state is obtained, hypercortisolism and alterations in the quiescent period disappear, indicating that these disturbances are “state” rather than “trait” dependent.214
In PTSD, some authors have reported that plasma cortisol levels are decreased in the afternoon and/or in the evening, and that the amplitude of circadian variations is enhanced.215–217 In fibromyalgia and in the chronic fatigue syndrome, cortisol levels have been reported to be low, normal, or elevated, depending on the study, but ACTH and cortisol pulsatility were found to be normal.218 In a well-documented study performed in constant routine conditions, normal circadian variations of plasma cortisol levels were evidenced in women with fibromyalgia.219
The Somatotropic Axis
The 24 Hour Profile of Growth Hormone in Normal Subjects
Pituitary secretion of GH is stimulated by hypothalamic GH-releasing hormone (GHRH) and is inhibited by somatostatin. In addition, the acylated form of ghrelin (acyl-ghrelin), a peptide produced predominantly by the stomach, binds to the GH-secretagogue receptor and therefore is another potent endogenous stimulus of GH secretion.220,221 In normal adult subjects, the 24-hour profile of plasma GH levels consists of stable low levels abruptly interrupted by bursts of secretion. The most reproducible pulse occurs shortly after sleep onset, in association with the first phase of SW sleep.222 Other secretory pulses may occur in later sleep and during wakefulness, in the absence of any identifiable stimulus. Studies in young male twins have evidenced a major genetic effect on GH secretion during waking, but not during sleep.223 In adult men, the sleep-onset GH pulse generally is the largest pulse observed over the 24-hour span. In normally cycling women, 24-hour GH levels are higher than in age-matched men, daytime pulses are more frequent, and the sleep-associated pulse, although still present in most cases, does not generally account for most of the 24-hour GH release.224 Typical profiles of young men and women are shown in Fig. 11-10. Well-documented studies have demonstrated that in women, the amplitude of GH secretory pulses is correlated with the circulating level of estradiol.224,225 In normally cycling young women, it also was observed that daytime GH secretion was increased during the luteal phase as compared with the follicular phase, and that this elevation correlated positively with plasma levels of progesterone but not estradiol.226
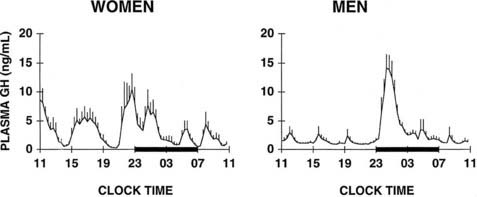
FIGURE 11-10. Mean (+standard error of the mean [SEM]) 24-hour plasma growth hormone profiles in nine men, age 18 to 30 years, and in seven women, age 21 to 33 years, during the follicular phase. The black bars represent the sleep periods.
(Adapted from Van Cauter E, Plat L, Copinschi G: Interrelations between sleep and the somatotropic axis. Sleep 21:533–566, 1998.)
Sleep onset will elicit a GH secretory pulse, whether sleep is advanced, delayed, interrupted, or fragmented.222 Thus, as illustrated in Fig. 11-3, shifts in the sleep-wake cycle are followed immediately by parallel shifts in GH rhythm.222 In night workers, the main GH secretory episode occurs during the first half of the shifted sleep period.227 The release of GH in early sleep is temporally and quantitatively associated with the amount of SW sleep.228,229 Both SW sleep and GH levels are increased during the recovery night following nocturnal total sleep deprivation as compared with the baseline predeprivation night, especially during the first 4 hours of sleep.230 Good evidence suggests that the mechanisms underlying the relationship between SW sleep and GH release involve synchronous activity of at least two different populations of hypothalamic GHRH neurons.231 Indeed, inhibition of endogenous GHRH by administration of a specific antagonist or by immunoneutralization inhibits both sleep and GH secretion.232 Additional evidence for the existence of a robust relationship between SW activity and GH release has been provided by studies using pharmacologic stimulation of SW sleep. Indeed, enhancement of SW sleep by oral administration of low doses of gamma-hydroxybutyrate (GHB), a natural metabolite of GABA used in treatment for narcolepsy, or of ritanserin, a selective serotonin (5-HT)2 antagonist, results in simultaneous and highly correlated increases in nocturnal GH secretion.108,233 Conversely, transient awakenings during sleep inhibit GH secretion.234 Thus, sleep fragmentation generally will decrease nocturnal GH release.
However, although sleep is clearly the major determinant of GH secretion in man, evidence for the existence of a circadian modulation of the occurrence and amplitude of GH pulses also has been found. This may reflect decreased somatostatin inhibitory activity in the evening and during the night,235 or it could result from the nocturnal rise in ghrelin, which occurs even in the absence of sleep.236 Thus, the major sleep-onset associated GH pulse is caused by a surge of hypothalamic GHRH coincident with a circadian period of relative somatostatin disinhibition.222,232 In normal-weight subjects, fasting, even for only 1 day, enhances GH secretion via an increase in pulse amplitude.237 Presleep GH pulses, reported by some investigators in normal men,238 may reflect the presence of a sleep debt, thus unmasking the circadian component of GH secretion.239 A recent study provides evidence for modulation of GH secretion by endogenous acyl-ghrelin, leading to higher GH peaks before times of food intake in subjects given regular meals.237
After a night of total sleep deprivation, a compensatory increase in GH release is observed during the daytime, so that the overall 24-hour secretion is not altered significantly.240 The mechanisms underlying this compensatory increase might involve decreased somatostatinergic tone and/or elevated ghrelin levels. Recurrent partial sleep restriction is associated consistently with the appearance of a presleep GH pulse.239
Aging is associated with dramatic decreases in circulating levels of GH (see Figs. 11-4 and 11-5).126,224 This reduction is achieved by a decrease in amplitude, rather than in frequency, of GH pulses.126,241,242 It also has been reported that the orderliness of GH secretion is decreased in the elderly.243 As illustrated in Fig. 11-5, this age-related GH decrease occurs in an exponential fashion between young adulthood and midlife and follows the same chronology as the decrease in SW sleep. Despite the persistence of high levels of sex steroids, plasma concentrations and pulsatile secretion rates of GH fall in midlife to less than half the values achieved in young adulthood. Thereafter, smaller and more progressive decrements occur from midlife to old age.129 Among the elderly, GH secretory profiles are similar in men and in women.224 The age-related reduction of GH secretion appears to result from increased somatostatin secretion and diminished GHRH responsiveness.244
It is interesting to note that during pregnancy, a placental GH variant, which substitutes for pituitary GH to regulate maternal insulin-like growth factor-1 (IGF-1) levels,245 is released in a tonic rather than a pulsatile fashion.246
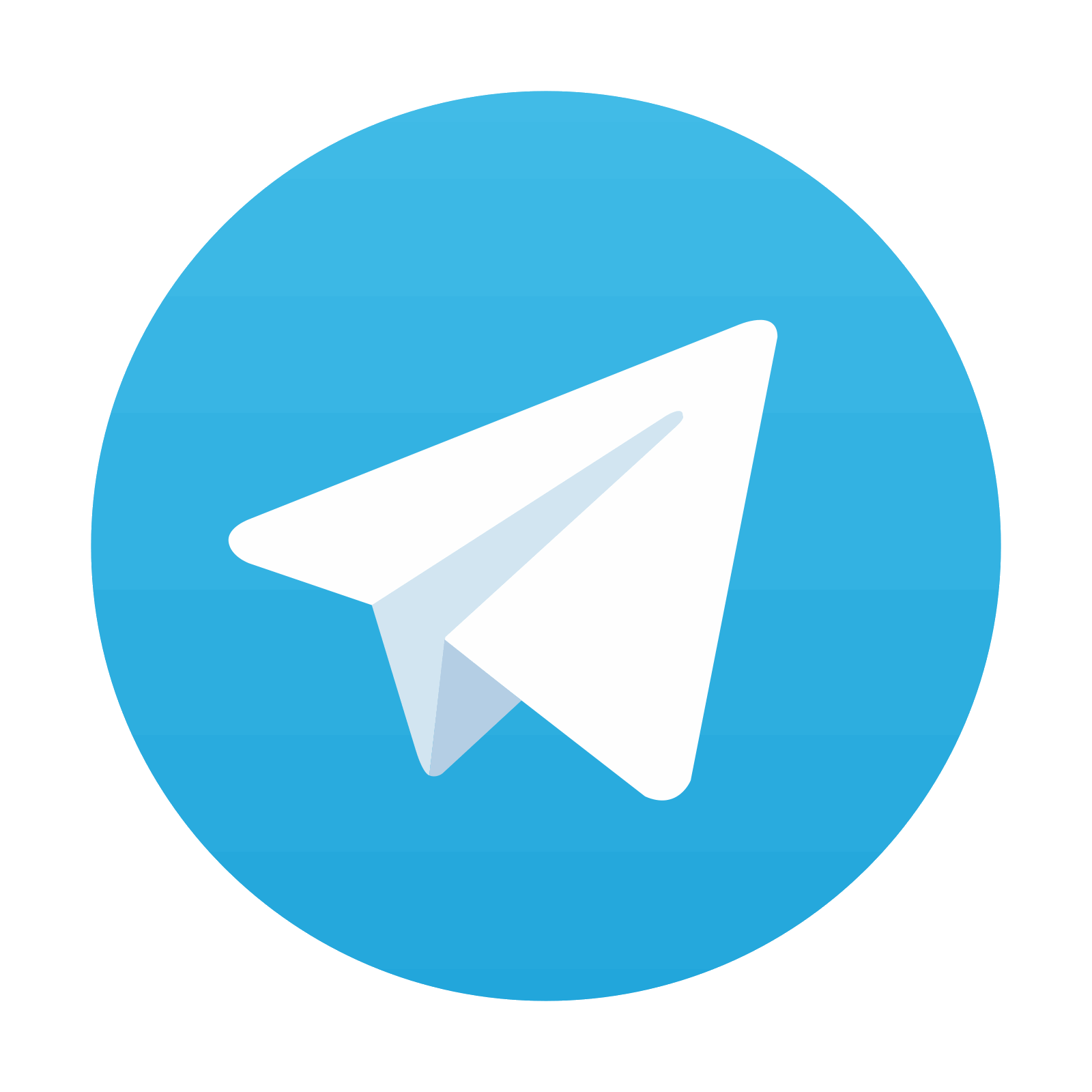
Stay updated, free articles. Join our Telegram channel
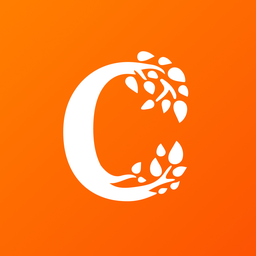
Full access? Get Clinical Tree
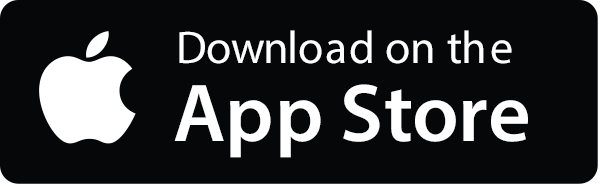
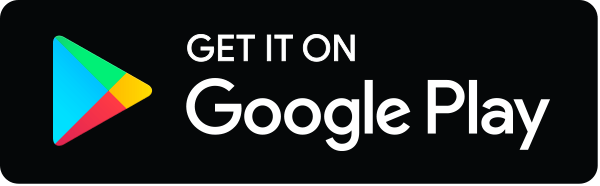