FIGURE 3-1. Schematic diagram of the anterograde secretory pathway in a peptide hormone–secreting endocrine cell. Secretory proteins synthesized in the endoplasmic reticulum (ER) are transported to and through Golgi stacks by vesicular transport. Within the trans-Golgi network (TGN), proteins are sorted to either constitutive or regulated secretory pathways. Immature secretory granules formed in the TGN are subject to additional sorting events during which clathrin-coated vesicles (dashes) divert constitutive membrane and soluble proteins back into the constitutive secretory pathway or to endosomes or Golgi. During exocytosis, mature secretory granules fuse with the plasma membrane, which is activated by increases in cytoplasmic calcium levels. Processing intermediates for a prepropeptide (such as preproinsulin) secreted by the regulated pathway are shown on the left and include cleavage of the N-terminal signal sequence (filled) in the ER and cleavage of the proregion (stippled) in the TGN-immature secretory granule stage.
By contrast, specialized secretory cells such as peptide hormone–secreting endocrine cells contain an additional pathway from the TGN to the cell surface, known as the regulated secretory pathway (see Fig. 3-1), which allows the acute regulated export of high concentrations of secretory proteins.5 In this pathway, proteins are sorted to dense-core vesicles or secretory granules that form by budding from the TGN with condensed luminal contents.5,6 Newly formed immature secretory granules may fuse with the plasma membrane in some endocrine cells.7,8 In addition, the immature secretory granules that form undergo further maturation, during which clathrin-coated vesicles form on the immature granule and sort out excess membrane and soluble contents for constitutive-like secretion or for recycling to the endosomal and Golgi compartment9–11 (see Fig. 3-1). Proteins that have been missorted to the immature granules (lysosomal enzymes) or that may function in maturation steps of granule-granule fusion (e.g., furin, synaptotagmin4, VAMP4, syntaxin6) are sorted from the immature granules back to the Golgi, which allows formation of fusion-competent mature secretory granules.11–14
Mature granules are stored in the cytoplasm for considerable periods ( > 10 hours) in the absence of stimulation,5,6 which enables endocrine cells to accumulate secretory products over an integrated period of biosynthetic activity. Endocrine cells accumulate a large number of secretory granules (Fig. 3-2), which can constitute 10% to 20% of the cellular volume and are filled with high (millimolar) peptide concentrations.15 Secretory granules discharge their contents only when an appropriate physiologic stimulus to the cell activates exocytotic fusion of the granule with the plasma membrane (discussed later), a process that is rapid (seconds to minutes) and mediated through rises in cytoplasmic calcium levels initiated through signal transduction events.16 Thus, an accumulated biosynthetic cargo can be rapidly discharged into the bloodstream at relatively high concentrations. The large size and condensed state of the contents of dense-core secretory granules are probably features of a specialized branch of the secretory pathway that coevolved with the development of an expanded circulatory system and the need to deliver adequate concentrations of signaling peptides into the bloodstream.
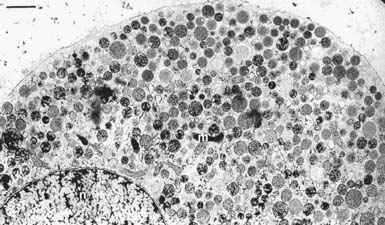
FIGURE 3-2. Transmission electron micrograph of a bovine adrenal medullary chromaffin cell prepared by cryofixation. Pleomorphic dense-core secretory granules with a mean diameter of 356 ± 91 (SD) nm are dispersed throughout the cytoplasm. Of approximately 22,000 granules per cell, very few (≈500) are in close proximity to the plasma membrane, possibly in a docked state. The scale bar corresponds to 1 µm.
(From Plattner H, Artalejo AR, Neher E: Ultrastructural organization of bovine chromaffin cell cortex-analysis by cryofixation and morphometry of aspects pertinent to exocytosis. J Cell Biol 139:1709–1717, 1997.)
Synthesis, Processing, and Sorting of Preprohormone Precursors
Secretory peptide precursors contain an N-terminal leader or signal peptide sequence to direct their synthesis in the ER and vectorial transfer into the cisternae of the ER-Golgi pathway17 (see Fig. 3-1). After transfer from the ER to the Golgi, most peptide hormone and neuropeptide precursors exist as prohormones from which multiple peptides are excised by proteolytic processing at sites usually marked by pairs of basic amino acid residues.18–20 The endoproteases responsible for precursor maturation belong to a prohormone convertase (PC) family of serine proteases related to bacterial subtilisin, which has several members20 (furin, PACE4, PC1, PC2, PC4, PC6A/B, LPC). PC1 and PC2, whose expression is restricted to tissues of neuroendocrine lineage, undergo sorting to dense-core vesicles formed in the TGN and are considered to be the proteases essential for the initial proteolytic maturation of neuropeptide and peptide hormone precursors.18–20 Although proteolytic cleavage of hormonal precursors may be initiated in the TGN,21 most of the cleavage occurs after entry into immature granules22 in the low-pH, high-calcium environment required for optimal PC activity (see Fig. 3-1). Mature secretory granules contain a “cocktail” of multiple peptides derived from a prohormone precursor that is discharged upon exocytosis, and the multiple bioactive peptides can exert concerted physiologic regulation.19,21,23 Sorting events in the immature granule also result in constitutive-like secretion of some of the peptide products (such as the C peptide of proinsulin).10,24 In some instances, mature peptides from a common precursor are segregated into distinct secretory granules, which may involve initial proteolysis before sorting in the TGN,21 or, alternatively, different mechanisms for sorting into dense-core vesicles.27 Production of distinct dense-core vesicles (e.g., those for prolactin and growth hormone in mammosomatotrophs) also can occur for proteins that are separate gene products.25
The biogenesis of immature secretory granules is closely linked to the condensation and sorting of prohormones in the TGN.9,19,21 Cellular mechanisms used for sorting to the regulated pathway appear to be common to neural, endocrine, and probably exocrine cell types as inferred from the finding that peptide hormone precursors, as well as pancreatic prozymogens expressed by DNA transfection, are properly sorted to the regulated pathway in neuroendocrine and exocrine cells.5,6 Because in many cases expressed protein chimeras containing prohormone sequences are properly targeted to the dense-core vesicles of the regulated secretory pathway in neuroendocrine cells, it is thought that prohormonal precursors contain sorting “signals” that are recognized by “sorting receptors” in the TGN (sorting by entry).27 Alternatively, sorting of dense-core vesicle constituents may occur by their selective retention in immature granules during post-Golgi sorting events that remove constitutive proteins from immature granules (sorting by retention).9,10 In either case, a common sorting motif for propeptides has not been identified nor has a common sorting receptor.
As recently summarized,27 several mechanisms are utilized to differing extents in different tissues for sorting specific prohormonal precursors or vesicle content protein. Precursors for adrenocorticotropic hormone, enkephalins, and insulin may contain a sorting signal that consists of similarly spaced acidic and hydrophobic residues on the surface of an amphipathic loop.30 Such regions were reported to interact with carboxypeptidase E, a hormone-processing enzyme that is membrane associated in secretory granules, which was proposed to be a sorting signal receptor.30,32 Aspects of this model have been discussed.24,27 In other studies, processing enzymes (carboxypeptidase E, PC 1/3 and 2) and other granule constituents (e.g., chromogranin A) were found to utilize α-helical regions that interact with cholesterol-rich membrane microdomains in the TGN for sorting to dense-core vesicles.27,31,33,39 The dibasic protease cleavage sites in some prohormone precursors may mediate sorting by interacting with the PCs that act at these sites.43 Last, a sorting-by-condensation model proposes that sorting in the TGN is mediated by protein aggregation that is promoted at low-pH and high-calcium concentrations in the TGN cisternae.29,34,35 Chromogranin B, an acidic granule protein ubiquitously expressed in neuroendocrine cells, aggregates under these conditions and also associates with membranes via a disulfide-bonded loop region of the protein that is required for proper targeting to regulated granules.26,28 Some evidence suggests that aggregation of chromogranin proteins in the TGN may suffice to promote the biogenesis of dense-core vesicles, although the mechanism is unclear.36–38 The property of some regulated (e.g., growth hormone, prolactin, follicle-stimulating hormone, PC2) but not constitutive (e.g., IgGs, albumin) secretory proteins to self-aggregate, as well as to aggregate heterophilically and to associate with membranes20,40 under TGN luminal conditions, provides the basis for the sorting-by-condensation model, which envisions prohormonal aggregates sorting away from constitutive secretory proteins by associating with specific membrane domains in the TGN.29,35 In summary, the diverse “signals” on soluble proteins that are sorted to the regulated secretory pathway consist of protein segments that interact with processing enzymes or with membrane lipid rafts, or regions that mediate aggregation and membrane association. The “receptors” that may decode these signals for sorting or retention consist of cholesterol-rich lipid rafts, prohormone processing enzymes, or granin proteins. By contrast, the targeting of transmembrane proteins to the regulated secretory pathway appears to be mediated largely by cytoplasmic sequences that interact with cytosolic protein factors such as clathrin adaptor proteins.41,42,46,50
For vesicle budding at several sites in the anterograde secretory pathway, transmembrane proteins link cargo in the vesicle lumen to the cytosolic components (e.g., coat proteins) required for vesicle formation, which provides a mechanism for coupling vesicle generation with content filling.44,45 It is unclear whether similar events occur during the formation of secretory granules in the TGN because potential cargo receptors and protein coats have not been identified. However, aspects of immature granule biogenesis in the TGN have been elucidated by studies of cell-free budding reactions.47–49,52,54 Granule formation in vitro requires adenosine triphosphate (ATP) and cytosolic protein factors. One of the required cytosolic factors is phosphatidylinositol transfer protein (PITP), which interacts with membrane phosphatidylinositol and to a lesser extent with phosphatidylcholine. PITP may alter the phospholipid composition of the Golgi membrane to facilitate budding54 or may promote the phosphorylation of phosphatidylinositol by a lipid kinase.48,55 The latter could account for the ATP dependence of vesicle formation. Phosphorylated inositides such as phosphatidylinositol monophosphate or bisphosphate (PIP or PIP2) are known to regulate membrane events by promoting protein (e.g., coat, cytoskeletal) recruitment to membranes56 or by serving as essential cofactors for membrane enzymes such as phospholipase D,57 which converts phosphatidylcholine to phosphatidic acid. The small guanosine triphosphate (GTP)-binding protein ARF1 (ADP [adenosine diphosphate] ribosylation factor), which is required for recruitment of coat protein to generate other Golgi-derived transport vesicles,44 is also required for secretory granule formation.49 ARF1 may function by recruiting an unidentified coat protein or cytoskeletal constituents, or by regulating the activity of a PIP2-dependent phospholipase D.51,52 Overall, the TGN-budding process that generates immature granules resembles other vesicle-budding events in their requirement for GTP-binding proteins and factors that alter membrane phospholipids.57 Immature secretory granules contain a type II PI 4-kinase and PIP, which may be required for subsequent maturation or priming events in preparation for fusion at the plasma membrane.59 Although the budding of immature secretory granules from the Golgi does not appear to involve clathrin recruitment, the immature granules contain missorted proteins from the Golgi (e.g., furin, carboxypeptidase D, syntaxin-6, VAMP-4, mannose-6-phosphate receptor) that are retrieved in a clathrin-dependent budding process that employs ARF-dependent recruitment of AP-1 and GGA (Golgi-associated, γ-ear–containing, ADP-ribosylation factor binding) proteins.53
Composition of Mature Secretory Granules
Mature secretory granules in endocrine and neural cells consist of a membrane bilayer that surrounds an electron-opaque dense core consisting of condensed secretory materials such as peptide hormones, granin proteins, and processing enzymes. In some endocrine cells, such as β cells in the islets of Langerhans, the contents are crystalline and consist of insulin hexamers chelated by zinc.60 Proteolytic processing of proinsulin in the immature granule is required to form this crystalline deposit in some species.61 Dense-core vesicles vary widely in properties from one endocrine cell type to another and range in size from 50 nm in the sympathetic nervous system to 200 nm in pituitary corticotrophs and gonadotrophs, and up to 1000 nm in pituitary mammotrophs or neurohypophyseal cells.
Mature secretory granules engage in multiple cellular functions, including vectorial transport of small molecules into the luminal space (nucleotides, divalent cations, protons, and neurotransmitters), translocation of the granules through the cytoplasm and their anchorage to cytoskeletal elements, docking of the granules at the plasma membrane, and their calcium-dependent exocytotic fusion at the plasma membrane. These functions would require an array of organelle-specific proteins exposed on the cytoplasmic face of the granule. Analyses of purified secretory granules have been undertaken to identify proteins that participate in aspects of the granule life cycle. The chromaffin granules of adrenal medullary tissue are best studied (see Fig. 3-2), although granules purified from anterior and posterior pituitary or from pancreatic islet cells also have been analyzed in some depth. Individual adrenal chromaffin cells contain 10,000 to 30,000 granules with a mean diameter of 350 nm,64,66 which has enabled extensive purification at yields of 2 to 3 mg per bovine adrenal gland.
The adrenal chromaffin granule possesses a number of general features likely to be representative of other secretory granules. Chromaffin granules consist of approximately 20% lipid and about 42% protein (percent dry weight). The membrane of the chromaffin granule exhibits a lipid composition similar to that of other cellular membranes but is notable for its relatively high cholesterol content, which is characteristic of late Golgi-derived membranes.67 In addition, a high concentration of lysophosphatidylcholine is present, which has also been reported for exocrine tissue granules but not for pituitary granules and synaptic vesicles.67 Thus, a high lysophospholipid content does not appear to be essential for a common granule function such as exocytotic fusion, but the precise role of lysophospholipids in granules is not known. Chromaffin (and other) granules contain, like many cellular membranes, 2% to 5% phosphatidylinositol, a phospholipid that is a precursor for the formation of PIP2, which is required in membrane fusion mechanisms (discussed later).
Although the characterization of chromaffin granule proteins was anticipated to identify constituents that mediate general functions of dense-core vesicles, including exocytosis, instead it mainly revealed specialized constituents unique to the function of these catecholaminergic and peptidergic granules.66 The composition of chromaffin granule protein is dominated by abundant proteins that catalyze catecholamine synthesis or the posttranslational processing of neuropeptides. About 75% of the protein is soluble in the lumen. Luminal contents are dominated by a family of acidic, heat-stable glycoproteins, the granins (chromogranin A and secretogranins I and II), and their proteolytic products. Granins may function in the aggregative sorting of peptide hormone precursors to the regulated pathway (discussed previously) and are general constituents of neuroendocrine secretory granules from the parathyroid, pituitary, thyroid, and pancreas, as well as of sympathetic neurons.68 Granins are also precursors for a variety of bioactive peptides such as pancreastatin, vasostatin, parastatin (derived from chromogranin A), and secretoneurin (derived from secretogranin II).64,66 Other chromaffin granule luminal proteins are glycoproteins (glycoprotein III), neuropeptides (enkephalins and neuropeptide Y), and enzymes for catecholamine synthesis (dopamine β-monooxygenase), neuropeptide proteolytic cleavage (carboxypeptidase E/H, PC1, and PC2), and peptide amidation (peptidylglycine α-amidating monooxygenase). Recent mass spectroscopic analysis of chromaffin granule soluble contents identified approximately 63 constituents, with the largest number representing prohormonal precursors (≈13) and proteases (≈9).58 The dense core of chromaffin granules observed by transmission electron microscopy (EM) is attributed to the high luminal content of granin proteins and neuropeptides in the millimolar concentration range.66 Small-molecular-weight constituents are also abundant and consist of catecholamines (≈0.6 M), ATP (≈0.15 M), ascorbic acid (≈0.02 M), and calcium (≈0.02 M). Other endocrine dense-core vesicles contain high concentrations of ATP and calcium.69
The membrane protein composition of chromaffin granules is dominated by membrane-bound dopamine β-monooxygenase and cytochrome b5, both dedicated constituents that function in the oxidation of dopamine to norepinephrine.66 Other membrane proteins found in lesser abundance are the subunits of the chromaffin granule proton pump (H+-ATPase), lysosome-associated membrane proteins (LAMP-1 and LAMP-2), and neuropeptide-processing enzymes that are present in soluble and membrane-anchored forms (PC1, PC2, carboxypeptidase E/H, peptidylglycine α-amidating monooxygenase).64 Molecular cloning with subsequent immunochemical detection also identified catecholamine transporters (vesicular monamine transporters VMAT1 and VMAT2) as chromaffin granule membrane constituents.70
A large number of more minor but functionally important membrane protein constituents have been identified immunochemically on chromaffin granules through the use of antibodies to proteins initially discovered on the compositionally simpler neuronal small clear synaptic vesicles (see Fig. 3-6). Several of these proteins, which are also found on other neural and endocrine dense-core vesicles, function in regulated exocytosis (synaptotagmin, synaptobrevin/VAMP [vesicle-associated membrane protein], Rab3A, cysteine string proteins). Proteins with putative regulatory roles (Go) or of unknown function (SV2, synaptophysin) have also been identified.72 A variety of Rab proteins (Rab3a, Rab27a, Rab14, Rab21, Rab35) and putative Rab-binding effector proteins (rabphilin, Slac2c/MyRIP, Slp4a/granuphilin) are present on granules, as are proteins that mediate actin-based granule translocation such as myosin V.74–77 Additional membrane constituents detected by activity include K channels,78 N-type Ca2 channels,79 and a phosphatidylinositol 4-kinase.80 Mass spectroscopic analysis indicated approximately 80 constituents on the chromaffin granule membrane, with considerable overlap with soluble constituents.58
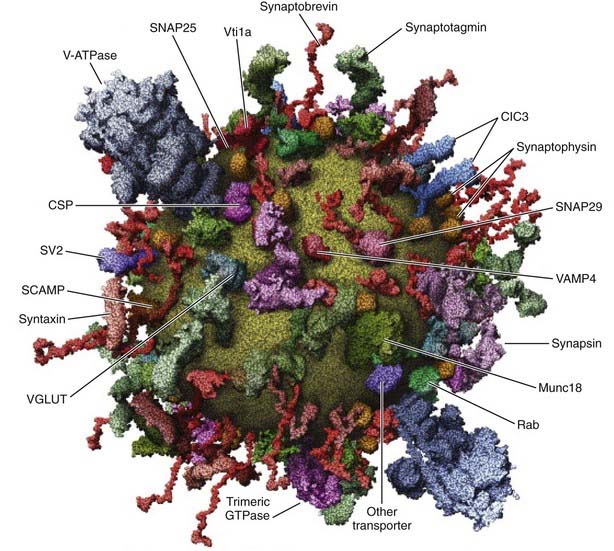
FIGURE 3-6. Membrane proteins associated with brain synaptic vesicles. Some of the characterized organelle-specific membrane proteins associated with synaptic vesicles are summarized in the figure. Synaptic vesicles, which are ≈40 nm diameter, are compositionally simpler than dense-core vesicles. Many of these proteins have also been identified on dense-core vesicles.
(Reprinted by permission from Takamori S, Holt M, Stenius K et al: Molecular anatomy of a trafficking organelle. Cell 127:831–846, 2006.)
Sequential Stages of the Regulated Secretory Pathway
In most endocrine cells, a majority of dense-core vesicles are cytoplasmic, with only a small portion in direct contact with the plasma membrane in a docked state (see Fig. 3-2). Dense-core vesicles undergo rapid translocation from their site of biogenesis in the TGN to sites in the cortical cytoskeleton, which occurs by kinesin-mediated movement on microtubules followed by myosin V-catalyzed transport via actin filaments.81,82 Docking of dense-core vesicles on the plasma membrane leads to a state of relative immobility,83 but there are multiple substates.62 Vesicle-plasma membrane interactions reported to mediate docking involve Rab27/rabphilin/SNAP-25,63 munc-18/Slp4a/syntaxin-1,65 and myosin V/syntaxin-181 complexes. The most recently arrived granules that dock at the plasma membrane are used for calcium-triggered exocytosis in preference to older granules, which are largely cytoplasmic.84 Peptide hormone secretion upon cellular activation is believed to proceed by the rapid exocytotic fusion of a portion of the docked granules (release-ready pool), which subsequently are replenished by recruitment of granules to the plasma membrane from a cytoplasmic recruitment pool.85 Thus, current views suggest a sequential pathway in which granules transit through recruitment, docking, and exocytotic fusion steps (Fig. 3-3).
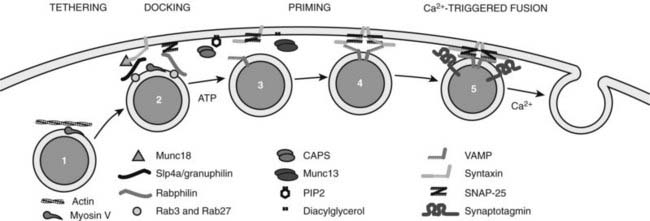
FIGURE 3-3. Late stages of dense-core vesicle exocytosis. The diagram depicts several stages through which secretory granules transit before fusion with the plasma membrane. 1, A recruitment pool of granules associated with cytoskeletal elements is recruited to the plasma membrane. 2, Granules are anchored close to and are docked at the plasma membrane by protein-protein interactions involving Rab3/27, rabphilin, SNAP-25 and Rab3/27, slp4a/granuphilin, and munc18. 3, An adenosine triphosphate (ATP)-dependent priming process involving the action of NSF on SNARE proteins and the synthesis of phosphatidylinositol 4,5-bisphosphate (PIP2) is required for granules to attain competence for calcium-triggered fusion. 4, The priming factors CAPS and munc13, interacting with PIP2 and diacylglycerol, respectively, act to promote intermediate SNARE complex formation possibly involving the zippering of trans SNARE complexes. 5, Calcium elevations to the 1- to 30-µM range trigger fusion in a process that requires synaptotagmin acting on SNARE complexes and the plasma membrane. Symbols depict several proteins essential at these stages of dense-core vesicle exocytosis.
Evidence for a sequential model is provided by rapid kinetic studies of exocytosis by patch clamp electrophysiologic methods, in which increases in membrane capacitance reflect expansion of the surface membrane area after exocytosis,87,88 and by amperometry studies, which use carbon fiber electrodes to detect secreted oxidizable granule constituents such as catecholamines.89 Capacitance increases and amperometric spikes from single-granule fusion events have been detected in adrenal chromaffin and other secretory cell types.87–90 Combining these techniques in a single pipette revealed that catecholamine content release can occur during transient reversible fusion of the granule with the plasma membrane.90 Cellular activation to elevate cytoplasmic calcium levels results in multiphasic increases in secretion (Fig. 3-4) that consist of at least two components—an ultrafast (or exocytotic burst) component within the first 100 msec, followed by a slower component over the ensuing 1 to 10 seconds. These components of exocytosis are interpreted to represent the sequential fusion of secretory granules in a docked release-ready state, followed by fusion of granules that require recruitment into the release-ready pool.87–91 The size of the exocytotic burst or release-ready pool (corresponding to 100 to 300 granules in chromaffin cells) is smaller than the number of docked granules (500 to 1000 granules in chromaffin cells; see Fig. 3-2) detected morphologically by EM, thus indicating that docked granules may exist in several functional states.83,87,92 The release-ready pool represents a very small fraction of the cellular granule complement of 10,000 to 30,000. Under physiologic stimulation conditions (i.e., splanchnic nerve stimulation), catecholamine secretion corresponding to 1% to 2% of the adrenal granule pool is mobilized, which indicates that the docked pool of granules in a release-ready pool is sufficient to mediate physiologic responses with short latency.93 Similar fractional release during physiologic stimulation is commonly observed in other endocrine tissues.94
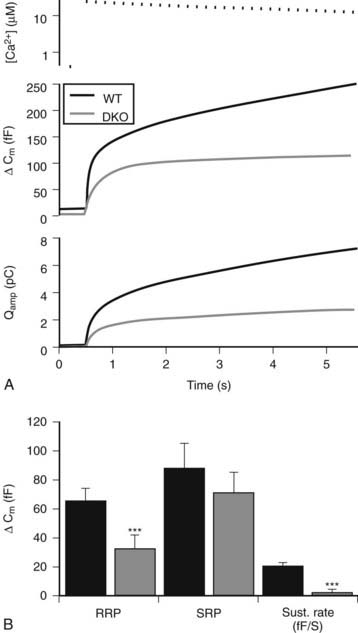
FIGURE 3-4. Multiple kinetic components of dense-core vesicle exocytosis in mouse chromaffin cells. Capacitance measurements with a patch clamp pipette in the whole-cell configuration (second panel in A) and amperometric current determinations with a carbon fiber electrode (third panel in A) were obtained simultaneously from a mouse adrenal medullary cell that was stimulated by elevating calcium levels to ≈27 µM (upper panel in A) by flash photolysis with a photolabile calcium chelator. A rapid (exocytotic burst) component and a slow component were detected, and the burst component was further kinetically resolved into a ready-release pool (RRP) and a slow-release pool (SRP). In this particular case, recordings were from wild-type and CAPS-1/CAPS-2 knockout mice. CAPS proteins were essential for maintaining the size of the RRP and and the sustained rate of exocytosis, which requires priming of recruited vesicles into the RRP (panel B).
(Taken from Liu Y, Schirra C, Stevens DR et al: CAPS facilitates filling of the rapidly releasable pool of large dense-core vesicles. J Neurosci 28:5594–5601, 2008.)
Recent technical developments have allowed the study of secretory granule movement in living neuroendocrine cells (Fig. 3-5). Fusion proteins consisting of prohormone peptides with green fluorescent protein at the carboxyl terminus undergo proper sorting to dense-core vesicles when expressed in neuroendocrine cells.95,96 Confocal fluorescence, or evanescent-wave microscopy, has enabled the tracking of individual granules during their cytoplasmic translocation, docking at the plasma membrane, and exocytosis.83,95–97 Granule movement to the plasma membrane is a directed process that occurs at speeds of approximately 50 nm/sec, followed by immobilization at the plasma membrane by a docking process that either occasionally reverses or culminates in exocytosis if calcium levels are elevated.83,96,97 New granules move to the plasma membrane and replenish the pool of docked granules within several minutes.77 Sustained stimulated secretion entails a cytoplasmic pool of mobile granules.95 Previous studies suggested that the actin cytoskeleton served as a barrier to the plasma membrane recruitment of granules, but more recent work indicates that granule recruitment to the plasma membrane is actin-mediated via myosin V, an actin-based motor that is present on secretory granules, which also may play a direct role in docking.77,81,82
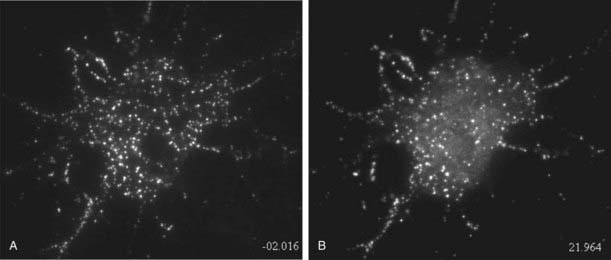
FIGURE 3-5. Exocytosis of dense-core vesicles in hippocampal cells recorded by evanescent-wave fluorescence microscopy. Cultured hippocampal neurons expressing ANF-EGFP were imaged by total internal reflection fluorescence microscopy to visualize the fluorescent vesicles in an optical section of about 200 nm. Images were captured before (left) and after (right) 20 seconds of depolarization with high K buffer. Following stimulation, a large number of dense-core vesicles in the soma of the neuron release ANF-EGFP, which is evident by a strong reduction in the number of fluorescent puncta or a reduction in their intensity. A general elevation of diffuse fluorescence near the cell represents released ANF-EGFP diffusing from sites of exocytosis. Evoked dense-core vesicle exocytosis in neurons is similar to that in endocrine cells.
(Taken from Xia X, Lessmann V, Martin TF: Imaging dense-core vesicle exocytosis in hippocampal neurons reveals long latencies and kiss-and-run fusion events. J Cell Sci, in press.)
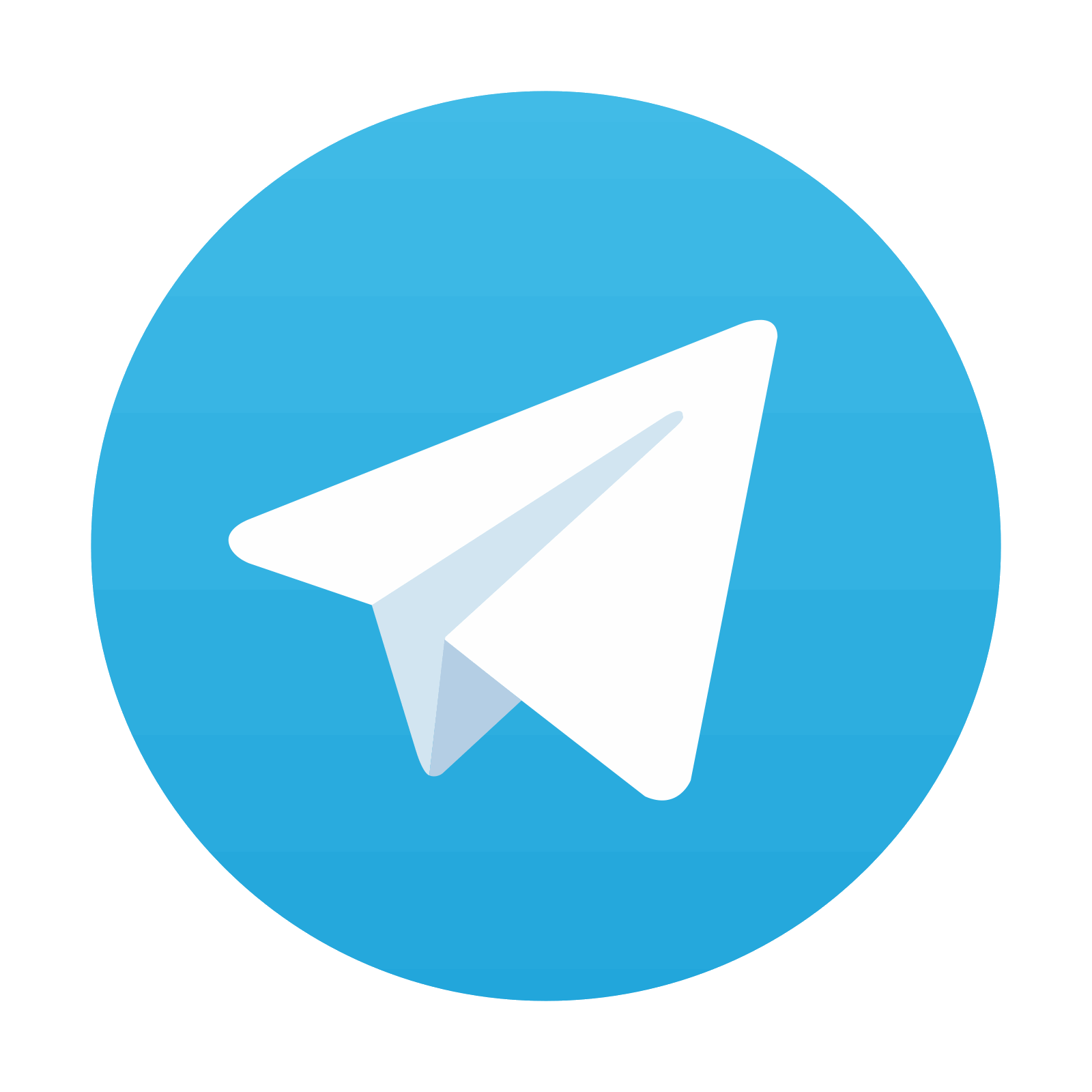
Stay updated, free articles. Join our Telegram channel
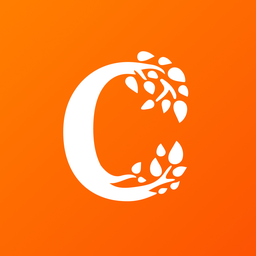
Full access? Get Clinical Tree
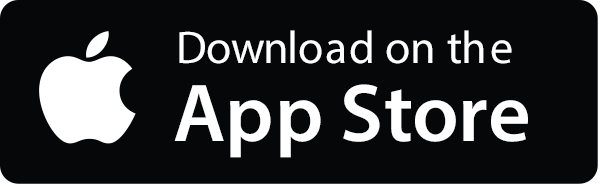
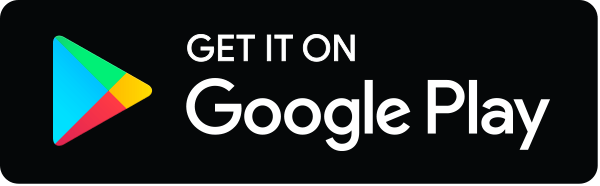