FIGURE 59-1. Stepwise schematic diagram of long bone development through endochondral ossification. Around embryonic day (E) 12 in mice, mesenchymal progenitor cells condense and differentiate into chondrocytes to form the cartilage anlagen that prefigure future long bones. Chondrocytes in the center become hypertrophic, while cells in the surrounding perichondrium differentiate into osteoblasts, forming a bone collar, the provisional cortical bone. The hypertrophic cartilage core subsequently is invaded by blood vessels and eroded and replaced by bone and marrow (primary ossification center [POC]). In the metaphysis, hypertrophic cartilage of the growth cartilage is continually replaced by trabecular bone, a process that relies on metaphyseal vascularization and mediates longitudinal bone growth. Around postnatal day (P) 5, epiphyseal vessels invade the avascular cartilage and initiate a secondary center of ossification (SOC). Discrete layers of residual chondrocytes form growth plates between the epiphyseal and metaphyseal bone centers to support further postnatal longitudinal bone growth. Ultimately (in humans), the growth plates close and growth stops. A detailed view of perinatal bone structure (boxed area) is provided in Fig. 59-2.
At this time in development (around embryonic day 14 to 15 in mice), the hypertrophic cartilage core becomes invaded by blood vessels. This process is accompanied by apoptosis of terminally differentiated hypertrophic chondrocytes, resorption of the calcified cartilage matrix by invading osteoclasts or related “chondroclasts,” and deposition of mineralized bone matrix on the remnants of calcified cartilage by perichondrium/periosteum-derived osteoblasts. The net result is replacement of the cartilage model by bone, vascular, and marrow elements: the primary ossification center (see Fig. 59-1). With the disappearance of the diaphyseal cartilage, the remaining chondrocytes, restricted to the opposing ends of the long bone, provide the engine for subsequent bone lengthening. This process is typified by precise temporal and spatial regulation of chondrocyte proliferation and differentiation, with the chondrocytes first flattening out and forming longitudinal columns of rapidly proliferating cells, and next, as they reach the ends of the columns closest to the center of the bones, maturing further to hypertrophic chondrocytes (Fig. 59-2). Finally, at the border with the metaphysis, the terminally differentiated chondrocytes undergo apoptosis, and the calcified hypertrophic cartilage matrix is replaced with cancellous or trabecular bone (primary spongiosa) in a process requiring progressive neovascularization by metaphyseal capillaries (see Fig. 59-1 and cellular details in Fig. 59-2). Thus, similar to the initial formation of the primary ossification center, endochondral bone formation at the growth cartilage involves rigorous coupling of vascular invasion with maturation and activity of chondrocytes, osteoclasts, and osteoblasts.
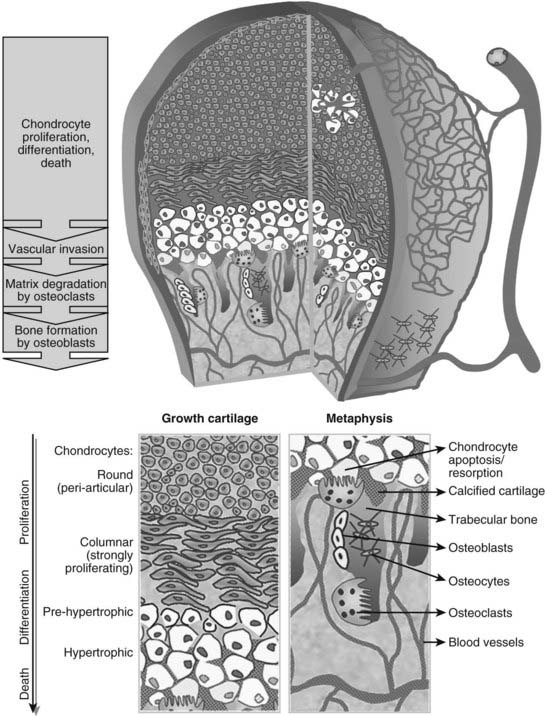
FIGURE 59-2. Schematic view of the cellular structure of developing long bones. The epiphysis is composed of chondrocytes, organized in layers of proliferation (round periarticular and flat columnar proliferating cells), progressive differentiation toward the metaphysis (pre-hypertrophic and hypertrophic chondrocytes), and cell death (apoptosis). The avascular cartilage is supplied by the epiphyseal blood vessel network that overlays its surface. In the metaphysis, blood vessels invading the terminal hypertrophic chondrocytes, osteoclasts resorbing the cartilage, and osteoblasts building bone on the cartilage remnants all act coordinately to replace the cartilage anlagen with bone and marrow.
At a certain time (around postnatal day 5 in mice), epiphyseal vessels, derived from the vascular network that overlays the cartilage tissue, invade the growth cartilage and initiate the formation of the secondary center of ossification. As a result, discrete layers of residual chondrocytes form true growth plates between the epiphyseal and metaphyseal bone centers, mediating further postnatal longitudinal bone growth. Ultimately, at least in humans, the growth plates completely disappear (close) at the end of adolescence in a process that actively requires the action of estrogen in both boys and girls, and growth stops. Remodeling of existing bone, replacing the primary spongiosa with lamellar bone in the secondary spongiosa and renewing the cortical bone, takes place throughout adult life, ensuring optimal mechanical properties of the skeleton and contributing to mineral ion homeostasis. This continual bone turnover is accomplished through the balanced action of osteoclasts and osteoblasts (see later) and results in a dynamic organization of honeycomb plate-like structures or trabeculae in the interior of the bone that are surrounded by blood vessels and bone marrow and housed within the cortical bone.
The mechanisms of embryonic bone development described here are largely recapitulated in the adult upon repair of bone defects. In contrast to soft tissues, which repair predominantly through the production of fibrous scar tissue at the site of injury, the skeleton possesses an astounding capacity to regenerate upon damage. As such, bone defects heal by forming new bone that is indistinguishable from adjacent, uninjured bone tissue. It has been appreciated for a long time that fracture repair in the adult bears close resemblance to fetal skeletal tissue development, with both intramembranous and/or endochondral bone formation processes occurring depending on the type of fracture. In recent years, this close resemblance has been supported by genetic and molecular studies showing that similar signaling pathways (see later) are at work in both settings,8,11–13 although additionally, some signaling molecules that are dispensable for development have been found to play essential roles in fracture repair.14,15
CELLULAR AND MOLECULAR CONTROL OF SKELETAL DEVELOPMENT
Our understanding of the regulatory mechanisms operating during skeletal development has grown tremendously over the past 20 years. Spontaneous mutations in humans and mice and experimental manipulation of genes by deletion or overexpression, causing loss- or gain-of-function, have identified many proteins involved in the differentiation of various cell types of bone and in the morphogenesis of individual bones. Here, we will review some of the principal growth and transcription factors and signaling cascades known to affect key aspects of bone development and maintenance, namely, chondrogenesis, vascular invasion of cartilage, resorption of cartilage and bone by chondroclasts/osteoclasts, and osteoblastogenesis.
Chondrogenesis
As outlined previously, a crucial step in endochondral bone development fundamentally entails the differentiation of cells in the mesenchymal condensations into chondrocytes. At least some of the mesenchymal cells are probably osteochondroprogenitor cells with the potential to differentiate into chondrocytes or osteoblasts. The decision to follow a given differentiation pathway is determined by the expression of key transcription factors, likely under the influence of canonical Wnt signaling. As will be discussed in greater detail later, Wnt signaling results in the stabilization of β-catenin, which then can act as a transcription factor and regulate the expression of downstream target genes. Recent studies have provided evidence that in the perichondrium, where cells are destined to become osteoblasts, Wnt signaling is high (at least in part induced by Shh),16 leading to high levels of β-catenin and inducing the expression of genes that mediate osteoblast differentiation (such as Runx2, a master regulator of osteoblastogenesis, see later), while inhibiting transcription of genes required for chondrocyte differentiation (such as Sox9). In the absence of β-catenin, these cells become chondrocytes instead of osteoblasts, as revealed via genetic modifications in mice. Conversely, in the inner region of the condensations, Wnt signaling must be low as these cells become chondrocytes.17–19 The initial conversion of mesenchymal progenitor cells into chondrocytes is driven by the transcription factor Sox9, whereas the combined action of Sox9, 5 and 6, is required to direct the subsequent differentiation of chondrocytes throughout all phases of the chondrocyte lineage.20–23
In the growth cartilage, the most extensively studied cartilage, differentiation of committed cells along the chondrocyte lineage characteristically gives rise to a stratified organization of small, round periarticular chondrocytes (also previously termed resting or reserve chondrocytes, because these cells proliferate only slowly after birth and can become columnar chondrocytes), flattened, columnar proliferating chondrocytes, and pre-hypertrophic and hypertrophic chondrocytes (see Figs. 59-2 and 59-3). Because progression of the chondrocytes through these stages is the driving force of actual bone development and growth, it is not surprising that the process is tightly controlled by a myriad of local signaling molecules, the best characterized being BMPs and transforming growth factor-β (TGFβ), FGFs, parathyroid hormone (PTH)-related protein (PTHrP), and Indian hedgehog (Ihh). The importance of these molecules is reflected by the fact that mutations in their receptors have been found to cause severe human dwarfing conditions, such as constitutive activating mutations of FGF receptor (FGFR)3 in human achondroplasia and thanatophoric dysplasia24–27 and PTH/PTHrP receptor mutations in Jansen and Blomstrand chondrodysplasia.28–30 Here, we will briefly review the basic mechanisms by which these signaling systems control the pace of proliferation and differentiation of growth chondrocytes.31–33
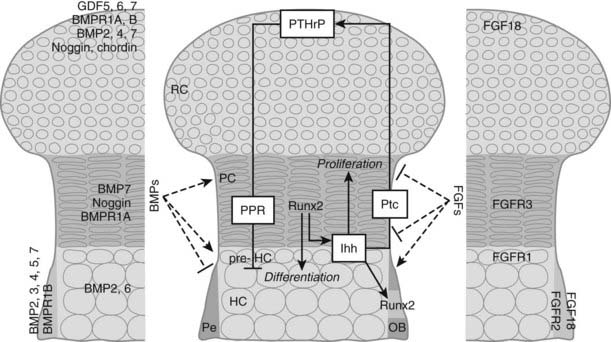
FIGURE 59-3. Regulation of chondrocyte proliferation and differentiation. Schematic representation of the epiphysis, with zones of periarticular round chondrocytes (RC), strongly proliferating columnar chondrocytes (PC), and (pre-)hypertrophic chondrocytes ([pre-]HC). Hypertrophic cartilage is surrounded by perichondrium (Pe), where osteoblasts (OB) develop. Centrally, the Indian hedgehog (Ihh)/parathyroid hormone-related protein (PTHrP)-negative feedback loop is indicated. Cells at the articular end of the bone secrete PTHrP, acting through the PTH/PTHrP receptor (PPR) on columnar chondrocytes to keep them in the proliferating pool and delay their differentiation. When the distance from the bone end becomes sufficiently large, cells escape the differentiation block and become Ihh-expressing pre-hypertrophic chondrocytes. In an as yet unknown manner, Ihh induces PTHrP expression. In addition, Ihh directly stimulates chondrocyte proliferation and converts perichondrial cells into OBs by inducing runt-related transcription factor 2 (Runx2), thereby determining the site of bone collar formation. Runx2 also favors Ihh expression and hypertrophic differentiation. Several bone morphogenetic protein (BMP) and fibroblast growth factor (FGF) family members are expressed at specific sites in the growth cartilage (left and right panels, respectively). BMPs and FGFs act antagonistically to modulate chondrocyte proliferation, Ihh expression, and terminal hypertrophic differentiation. GDF, Growth and differentiation factor; Ptc, patched; BMPR, BMP receptor; FGFR, FGF receptor.
BMPs, secreted proteins belonging to the TGFβ superfamily, transduce signals through serine/threonine kinase receptors, composed of type I and II subtypes, activating intracellular proteins of the Smad family that relay the BMP signal to target genes in the nucleus.34 BMPs were first discovered as agents in bone matrix capable of inducing ectopic formation of cartilage and bone after injection into subcutaneous tissues.35–38 Subsequent studies have shown that BMPs, some of which are also called growth and differentiation factors (GDFs) are, in fact, signaling proteins crucial for regulating development in almost all the principal organs and tissues.39,40 In the morphogenesis of the skeleton, BMPs have been implicated in early limb patterning, as well as in the subsequent processes of chondrogenesis and osteogenesis. Initially, the role of BMPs in the correct formation of mesenchymal condensations was highlighted by the mutation of the BMP5 gene in the mouse skeletal mutant short ear (se)41 and by abnormalities observed in brachypodism (bp) mice that were attributed to mutations in the GDF5 gene.42 In both se and bp mice, the abnormalities were traced back to altered size or shape already apparent in the mesenchymal condensations of the respective skeletal elements that were affected. More recently, studies in which two BMP type I receptors were ablated at the condensation stage demonstrated that BMP signaling is essential for converting condensing mesenchyme into chondrocytes; the mutant cells expressed undetectable amounts of the essential Sox9, L-Sox5, and Sox6 transcription factors.43 Similarly, when the potent BMP inhibitor noggin was introduced in early-stage mouse or chick limbs, mesenchymal condensation and chondrogenesis did not take place.44,45 BMPs also play critical roles at later stages of cartilage development. These functions have been difficult to document in vivo because in some cases, inactivation of specific BMPs led to severe early defects and lethality (e.g., BMP-2, BMP-4), precluding investigation of the later stages; in other cases, removal of individual family members (such as BMP-7) displayed no defects in skeletogenesis, presumably because of functional redundancy between the various BMP ligands and receptors (around 20 BMP family members have been identified to date). Further, various models have yielded superficially contradictory results, presumably reflecting the multiple actions of BMPs and the interactions of BMP signaling with other pathways. Recent and ongoing studies therefore employ conditional (site-specific) and/or combined (multiple targets) mutagenesis strategies. As such, it was shown in vivo that BMP signaling (through type I receptors on chondrocytes) positively regulates chondrocyte proliferation and survival, while concomitantly delaying the conversion to terminal hypertrophic differentiation,46 confirming previous limb culture results.47,48 These effects likely involve interactions between the BMP, FGF, and Ihh signaling pathways (see later); indeed BMP signaling seems to antagonize FGF actions and to promote Ihh expression. Studies in which BMP-2 and BMP-4 were ablated in limb development also revealed an essential role of BMP signaling in osteoblast differentiation (see later).49 It is not surprising that perichondrium, chondrocytes, and osteoblasts express multiple BMPs, BMP receptors, and BMP antagonists to regulate this essential pathway (see Fig. 59-3).
The FGF pathway similarly involves multiple ligands and receptors in regulating skeletal development.50 FGF1851,52 and FGF953 appear to be the most important FGF ligands in regulating chondrogenesis identified so far. These ligands activate FGFR3 expressed on proliferating chondrocytes. Activating mutations in the FGFR3 gene cause dominantly inherited human dwarfing chondrodystrophies due to impaired chondrocyte proliferation,24 and FGFR3 inactivation in mice increases chondrocyte proliferation and prolongs growth.54,55 This finding was surprising given that FGFs in most tissues act as potent mitogens; although this may pertain in early chondrogenesis, in which FGF18 stimulates proliferation,56 later in development, signaling through FGFR3 constitutes a master block on chondrocyte proliferation through activation of STAT1, which activates the cell cycle inhibitor p21Waf1/Cip1.57–59 In addition, although previous in vitro experiments had indicated that FGF signaling accelerates the late steps of chondrocyte hypertrophy,47 mouse models of achondroplasia and thanatophoric dysplasia have shown delayed hypertrophic differentiation of chondrocytes, strongly suggesting that FGFR3 signaling in vivo inhibits chondrocyte differentiation.25,60–63 This effect is relayed by activation of the mitogen-activated protein kinase (MAPK) pathway in chondrocytes, which affects longitudinal growth by regulating hypertrophic chondrocyte differentiation and matrix deposition.64–66 In vitro and in vivo experiments suggest that snail1 is required downstream of FGFR3 signaling for regulating its effects on chondrocyte proliferation and differentiation through activation of the Stat1/p21 and MAPK/Erks pathways, respectively.67 Yet, the effects of FGFR3 are regulated only in part by direct signaling in chondrocytes, and in part indirectly by modulating the expression of the Ihh/PTHrP/BMP signaling pathways. Indeed, mice harboring an activating mutation in FGFR3 have decreased expression of Ihh and its receptor and downstream target Patched (Ptc), and of BMP4, whereas in mice lacking FGFR3, Ihh, Ptc, and BMP4 expression are upregulated.60,68,69 During chondrocyte differentiation, the FGF and BMP pathways generally appear to antagonize each other,46 and both FGF signaling and BMP signaling regulate Ihh production (see Fig. 59-3). Thus, each of these pathways has multiple mechanisms for communicating with each other as they regulate chondrocyte and osteoblast differentiation.
A major regulatory system in growth chondrocytes is provided by Ihh, a member of the conserved family of hedgehog proteins, and PTHrP.32 The Ihh/PTHrP pathway forms a negative-feedback loop that regulates the onset of hypertrophic differentiation (see Fig. 59-3). Ihh is produced by prehypertrophic and early hypertrophic chondrocytes and signals through its receptor Ptc to stimulate expression of PTHrP by chondrocytes located near the periarticular ends of bone. Increased production of Ihh or production of Ihh closer to the cells making PTHrP leads to increased PTHrP production. PTHrP in turn signals back to its receptor—the PTH/PTHrP receptor that also responds to PTH in osteoblasts and kidney—that is expressed at low levels by proliferating chondrocytes and strongly by prehypertrophic cells. This signaling slows down chondrocyte differentiation and keeps chondrocytes in the proliferative state (see Fig. 59-3). This slowed differentiation consequently delays the generation of cells that can produce Ihh; therefore, the production of PTHrP is lowered. Thus, a negative-feedback signaling pathway allows PTHrP and Ihh to regulate each other and, in turn, to control the pace of chondrocyte development in the growth plate.32,70,71 It is important to note that the phenotype of the Ihh-/- mice72 revealed that Ihh has additional functions in endochondral bone formation, independent of regulation of PTHrP production. Indeed, these mutant mice also displayed a marked decrease in chondrocyte proliferation and absence of mature osteoblasts and bone collar formation.72 The regulation of chondrocyte proliferation represents a direct action of Ihh signaling to chondrocytes.73,74 Ihh also accelerates the conversion of round periarticular chondrocytes to flat columnar chondrocytes.75 Control of bone collar formation is mediated by Ihh-induced direct actions on perichondrial cells that are probably bipotential osteochondroprogenitors, and that are driven into the osteoblast lineage under the influence of Ihh stimulating the expression of Runx2. Runx2, an essential transcription factor in osteoblast development (see later), conversely also regulates Ihh expression and hypertrophic differentiation.72,76–79 From this, it is evident that Ihh is a master regulator of endochondral bone development, coordinating chondrocyte proliferation, chondrocyte maturation, and osteoblast differentiation (see Fig. 59-3). Recently, a continued role for Ihh in the postnatal growth plate has been revealed by employing inducible mutagenesis in mice.80
Aside from the above mentioned factors, chondrogenesis is affected by several other growth factors, cytokines, and hormones, including growth hormone (GH) and insulin-like growth factors (IGFs), C-natriuretic peptide, retinoids, thyroid hormone, estrogen, androgen, 1,25-dihydroxyvitamin D3 [1,25(OH)2D3], glucocorticoids, and others.32,81
Importantly, a main difference between mesenchymal condensations that differentiate toward the osteoblast lineage in and becoming intramembranous bones, and endochondral cartilaginous condensations is that the latter typically develop over a prolonged period in the absence of blood vessels. As an avascular tissue, cartilage consequently faces the challenge of hypoxia.82,83 The main mediator of cellular responses to hypoxia is the transcription factor hypoxia-inducible factor (HIF).84 Recently, HIF1α was shown to influence chondrocyte differentiation and to be required for survival of chondrocytes, because mice with conditional inactivation of HIF1α displayed aberrant apoptosis of chondrocytes located in the center of the growth cartilage, farthest from blood vessels.85,86 The precise mechanisms by which HIF1 prevents chondrocyte apoptosis have yet to be fully explained. Part of the explanation may include an indirect survival effect of HIF1, mediated through induction of vascular endothelial growth factor (VEGF), as deletion of VEGF from cartilage also resulted in the apoptotic phenotype.87,88 Alternatively, and not mutually exclusively, HIF1 may facilitate a vital switch in the metabolism of hypoxic chondrocytes by inducing the anaerobic glycolytic pathway,86,89–91 and thereby allow their further differentiation up to the hypertrophic stage, when the chondrocytes induce vascular invasion (see next).
Vascular Invasion of Cartilage
Both temporally and spatially, chondrocyte differentiation is followed by vascular invasion of the terminally differentiated, hypertrophic cartilage. This step is an absolute requirement for endochondral bone development and growth to proceed, as physical blockage of blood vessel invasion into the hypertrophic cartilage of fetal skeletal explants completely halts their development,92 and blocking the blood supply of bone in vivo results in reduced longitudinal growth.93,94 The importance of skeletal vascularization goes beyond endochondral bone development. In fact, as a rule, any type of bone formation occurs in close spatial and temporal association with vascularization of the ossified tissue. The reasons that the vascular system is crucial for bone development, maintenance, and repair obviously include its intrinsic function to supply oxygen, nutrients, and growth factors/hormones to bone cells as required for their specified activities. In addition, the blood vessels serve to bring in (precursors of) osteoclasts that will degrade the cartilage or bone extracellular matrix, to remove end products of the resorption processes, and to bring in progenitors of osteoblasts that will deposit bone.95–97
During endochondral ossification, the initially avascular cartilage template is replaced by highly vascularized bone and marrow tissue through three consecutive vascularization events (see Fig. 59-1). First, initial vascular invasion of the cartilage anlagen during embryonic development (sometimes called quiescent angiogenesis) involves endothelial cells invading from the perichondrial tissues and organizing into immature blood vessels in the primary center of ossification. Second, capillary invasion at the metaphyseal border of the growth cartilage mediates rapid bone lengthening. Third, vascularization of the cartilage ends initiates the formation of secondary ossification centers (see Fig. 59-1). Although the vessel systems associated with the different stages of cartilage vascularization are believed to be substantially different,98,99 a common feature is that invasion of cartilage at all times is preceded by chondrocyte hypertrophy. Immature chondrocytes are resistant to vascular invasion because of the production of angiogenic inhibitors, such as chondromodulin-I, troponin-I, and thrombospondins.100–103 In contrast, when chondrocytes become hypertrophic, they switch to production of angiogenic stimulators, thereby becoming a target for capillary invasion and angiogenesis.104,105 One exceptionally potent angiogenic stimulator, VEGF, has received much attention, as it is expressed at very high levels by hypertrophic cartilage, as well as by osteoblasts and osteoclasts. Although the regulation of VEGF expression is still largely unresolved and may vary depending on the developmental stage and specific location and cell type, several mechanisms have been implicated to date. Hypoxia seems to be a crucial trigger of VEGF expression in chondrocytes, osteoblasts, and possibly osteoclasts, through mechanisms that involve HIF both in vitro106–109 and in vivo.110 During early bone development, VEGF transcription may be induced by Runx2 (see later), given that expression of VEGF was impaired in Runx2-deficient mice and no blood vessel ingrowth into the cartilage occurred.111 In addition, several hormones [including PTH, GH, and 1,25(OH)2D3] and locally produced growth factors (e.g., FGFs, TGFβ, BMPs, IGFs, platelet-derived growth factor [PDGF]) have been demonstrated to be involved, at least in vitro, in the regulation of VEGF expression.112
Important insight into the crucial physiologic function of VEGF-mediated angiogenesis in bone was first provided by Gerber et al.,113 who inhibited VEGF action in juvenile mice through administration of a soluble VEGF receptor chimeric protein (sFlt-1). VEGF inhibition impaired vascular invasion of the growth plate, and concomitantly, trabecular bone formation and bone growth were reduced and the hypertrophic cartilage zone became enlarged, likely as the result of reduced osteoclast-mediated resorption (see later).113 Additional mouse genetic studies performed over the past decade have univocally demonstrated that VEGF is an essential mediator of all three key vascularization stages of endochondral bone development. Although a mouse with the VEGF gene ablated in all cells could not be employed owing to early lethality of even heterozygous VEGF knockout embryos, several alternative mutagenesis approaches have been and are being exploited to investigate the role of VEGF in bone. These models include Cre/LoxP-mediated conditional inactivation of the VEGF gene (VEGFa) in type II collagen–expressing chondrocytes, and expression of only one of the three major VEGF isoforms by genetically engineered mice.87,88,114–116 Altogether, these models have exposed multiple essential roles of VEGF and its isoforms in endochondral ossification, not only as a key inducer of vascularization but also as a direct modulator of bone development by affecting the various cell types involved. Perichondrial cells, osteoblasts, and osteoclasts are well documented to express several VEGF receptors and to respond to VEGF signaling through enhanced recruitment, differentiation, activity, and/or survival.112,117,118 These pleiotrophic actions of VEGF on various cells in the bone environment may contribute to the tight coordination of vascularization, ossification, and matrix resorption that is characteristically seen in endochondral ossification. Indeed, the current model is that VEGF secretion in the bone environment will attract blood vessels and stimulate endothelial cells to form new blood vessels, which will be indirectly associated with increased potential delivery of osteoblast and osteoclast progenitors.81 At the same time, VEGF signaling directly stimulates the recruitment and differentiation of osteoblasts to form bone.112,114,119,120 VEGF works as a chemoattractant stimulating osteoclast invasion of cartilage, and enhances osteoclast differentiation, survival, and resorptive activity.121–127 A positive-feedback system is likely established, as chondroclast/osteoclast-derived matrix metalloproteinase (MMP) 9 may release more matrix-bound VEGF from the cartilage that is being resorbed (Fig. 59-4).81,128
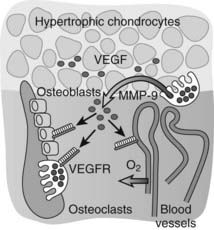
FIGURE 59-4. Vascular invasion and the role of vascular endothelial growth factor (VEGF). VEGF is produced at high levels by hypertrophic chondrocytes and is sequestered in part in the cartilage matrix upon its secretion. Trapped VEGF can be released from the matrix by proteases such as matrix metalloproteinase (MMP) 9 secreted by osteoclasts/chondroclasts during cartilage resorption. VEGF then can bind to its receptors (VEGFRs) on endothelial cells and stimulate the guided attraction of blood vessels to invade the terminal cartilage. Osteoblasts and osteoclasts also express VEGF receptors, and VEGF can affect their differentiation and function both directly and indirectly by enhancing metaphyseal vascularization, thus playing a coordinative role in the key events that mediate bone development and growth.
Osteoclastogenesis and Skeletal Tissue Resorption
At the time of vascular invasion of hypertrophic cartilage, the chondrocytes undergo apoptosis, and remaining cellular debris and matrix components become degraded and are digested by co-invading osteoclasts (or related cells termed chondroclasts that have been postulated in the context of resorption of cartilage128) through their proteolytic enzyme products.81 Beyond development, the osteoclast remains a key participant in the regulation of bone mass, as bone is constantly being remodeled throughout life (see later). Several pathologic conditions are due to an imbalance between bone formation and resorption, most frequently involving excessive osteoclast activity. Such skeletal diseases include osteoporosis, a common low bone mass disorder typically prevalent in postmenopausal women, as well as periodontal disease, rheumatoid arthritis, multiple myeloma, and metastatic cancer. On the other hand, osteopetrosis, a rare human disease characterized by increased bone mass and obliteration of the bone marrow cavity, is caused by impaired osteoclast differentiation and/or function. Many efforts have been made to dissect the regulatory pathways leading to functional osteoclasts.129–134
Osteoclastogenesis
Osteoclasts are giant multinucleated cells that have the unique capacity to efficiently degrade mineralized tissues. Osteoclasts are derived from hematopoietic precursor cells of the myelomonocytic lineage and share a common precursor with macrophages.131 Recognition of the intertwining control and close interactions of the immune and skeletal systems has led recently to the emergence of the novel integrating field of osteoimmunology.131,132 Early differentiation of the bipotential macrophage/osteoclast precursor cells is regulated by PU.1, a versatile hematopoietic cell-specific transcriptional regulator of the ETS family of transcription factors. The commitment to macrophages/osteoclasts is dependent on a high level of activity of PU.1131,135–137 (Fig. 59-5); PU.1 regulates the lineage fate decision of early progenitors by directly controlling expression of the c-Fms gene, which is a key determinant of differentiation into the macrophage/osteoclast lineage (see later).138,139 Consequently, PU.1-null mice lack both macrophages and osteoclasts, and are osteopetrotic.136,140,141 PU.1 also regulates the transcription of another key osteoclastogenesis control gene, encoding receptor activator of nuclear factor-κB (RANK) (see later) in myeloid progenitors.142 Although mature macrophages and osteoclasts have some cell surface markers in common, the latter express high levels of tartrate-resistant acid phosphatase (TRAP), cathepsin K, vitronectin, calcitonin receptor, and αvβ3 integrin, which typify the osteoclast lineage (see later).81
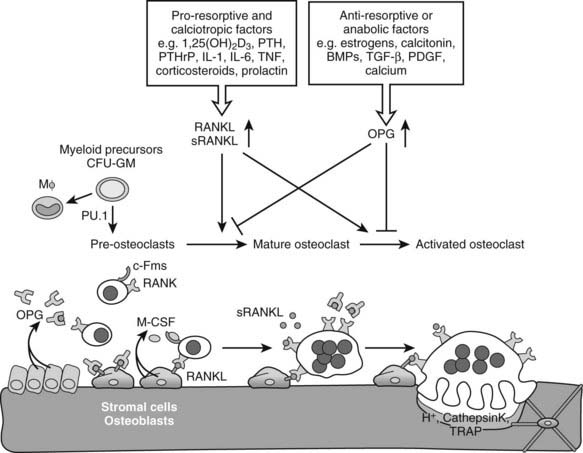
FIGURE 59-5. Osteoclastogenesis. The transcription factor PU.1 is indispensable for development of the osteoclast and macrophage (Mφ) lineages from a common myeloid precursor. Macrophage-colony stimulating factor (M-CSF) acting upon the receptor c-Fms is required for the conversion of the progenitor to become a pre-osteoclast. Signaling through the membrane-bound receptor activator of nuclear factor-κB (RANK) promotes further differentiation of pre-osteoclasts to mature polykaryotic osteoclasts and their subsequent activation to bone-resorbing cells that secrete protons and lytic enzymes. RANK signaling is induced by receptor activator of nuclear factor-κB ligand (RANKL), present on osteoblasts and bone marrow stromal cells, as well as on lymphocytes and possibly in a soluble form in serum. The soluble antagonist osteoprotegerin (OPG) competes with RANK for RANKL binding, thereby functioning as a negative regulator of osteoclast differentiation, activation, and survival. RANKL expression is induced by pro-resorptive and calciotropic factors that stimulate osteoclastogenesis. Conversely, OPG is induced by factors that block bone catabolism and promote anabolic effects.
After the precursor cells have committed to the osteoclast lineage, they are subjected to a complex multistep process that culminates in the generation of mature, multinuclear, activated osteoclasts; these steps include proliferation, maturation, and fusion of differentiating precursor cells, and finally activation of resorption (see Fig. 59-5).81,130,132,134 Two critical cytokines are essential for osteoclastogenesis: macrophage-colony stimulating factor (M-CSF) and receptor activator of nuclear factor-κB ligand (RANKL) (also known previously as osteoprotegerin ligand [OPGL], osteoclast differentiation factor [ODF], or tumor necrosis factor [TNF]-related activation-induced cytokine [TRANCE]). Both of these signaling molecules are strongly expressed by bone marrow stromal cells (i.e., osteoblast progenitors) and osteoblasts; M-CSF is produced in both a soluble and a membrane-bound form, and RANKL is made exclusively as a membrane protein by osteoblasts. The process of osteoclastogenesis requires direct cell-to-cell interaction between stromal/osteoblastic cells and osteoclast precursors, presumably because of these key membrane-bound ligands (see Fig. 59-5). Both PTH and 1,25(OH)2D3 and several other osteotropic factors stimulating resorption increase the expression of RANKL on stromal/osteoblastic cells130,143,144 (see later). Moreover, before the identification of RANKL, in vitro investigation of osteoclastogenesis relied on contact-dependent co-culture of osteoclast progenitors with stromal/osteoblastic cells stimulated with PTH or 1,25(OH)2D3. Now we know that RANKL, together with M-CSF, is sufficient to induce in vitro osteoclast differentiation from spleen- or bone marrow–derived precursors in the complete absence of stromal/osteoblastic cells.145 Furthermore, not only osteoblasts but also chondrocytes146–148 and T cells149,150 synthesize and secrete RANKL and are able to support osteoclastogenesis. This may be important physiologically during osteoclast differentiation and invasion at the hypertrophic cartilage during endochondral bone development and growth, and it definitely plays a major role pathologically. For instance, production of RANKL by T cells has been implicated as an activator of osteoclastic resorption in inflammatory mediated bone and cartilage destruction, as is seen in several autoimmune disorders, including rheumatoid arthritis,81,130,151 likely working synergistically with TNFα.152,153
M-CSF and RANKL affect several steps of the osteoclastogenesis cascade by binding to their respective receptors, c-Fms and RANK, which are expressed by osteoclast progenitors and osteoclasts at all stages of differentiation. M-CSF signaling is essential early on in the lineage for proliferation, differentiation, and survival of osteoclast/macrophage precursors. The essential roles of M-CSF were illustrated through analysis of mice carrying the op/op mutation, an inactivating point mutation in the M-CSF gene; these mice have osteopetrosis with low numbers of macrophages and a complete lack of mature osteoclasts.154–157
RANKL is an essential inducer of multiple aspects of osteoclastogenesis, including osteoclast differentiation, fusion, activation of mature osteoclasts to resorb mineralized bone, and survival.158–161 Through these actions, RANKL potently stimulates bone resorption. RANKL acts by binding to its signal transducing receptor, RANK, a member of the TNF receptor family present on pre-osteoclasts and mature osteoclasts. Consistent herewith, an activating mutation of the human RANK gene was found in patients with familial expansile osteolysis, a disease of excess bone resorption.162 Recent genetic and cell biological studies have begun to elucidate the complex signaling cascade downstream of RANK/RANKL.130,163 Briefly stated and oversimplified, the binding of RANKL to RANK on the surface of osteoclast precursors recruits the adaptor protein TNF receptor–associated factor (TRAF) 6 to the cytoplasmic domain of RANK, leading to activation of NF-κB and its translocation to the nucleus. NF-κB increases c-Fos expression, and c-Fos, as a component of the activator protein (AP)-1 complex, interacts with the master transcription factor for osteoclastogenesis, nuclear factor of activated T cells c1 (NFATc1), to induce osteoclast-specific genes (such as the genes encoding TRAP and calcitonin receptor; see later).164–167
The actions of RANKL are regulated negatively by a secreted soluble decoy receptor termed osteoprotegerin (OPG) (previously also known as osteoclastogenesis inhibitory factor [OCIF]), also a member of the TNF receptor superfamily. OPG sequesters RANKL molecules and thereby blocks their binding to RANK.130,168 As such, OPG protects bone from excessive resorption; this conclusion is supported by the finding that certain homozygous deletions of OPG in humans can cause juvenile Paget’s disease, a disorder characterized by increased bone remodeling, osteopenia, and fractures.169 The relative concentrations of RANKL and OPG in bone thus are major determinants of bone mass and strength. OPG is widely expressed; it is not surprising that its expression by osteoblasts and stromal cells is positively regulated by bone anabolic or antiresorptive factors, such as estrogen and calcitonin.130
The essence of the RANK/RANKL/OPG cascade was further clarified by mouse genetic studies. Mice lacking RANKL170 or RANK171,172 and mice with increased circulating OPG by transgenic overexpression173 were severely osteopetrotic owing to a block in osteoclastogenesis. Conversely, targeted mutagenesis of OPG,174,175 overexpression of an sRANKL transgene,176 and administration of RANKL159,160 in mice all led to increased osteoclast formation, activation, and/or survival and resulted in an osteoporotic phenotype. In summary, RANKL and OPG act in an antagonistic fashion to regulate bone resorption, and their respective expression levels are under the control of proresorptive and antiresorptive factors, including several hormones, cytokines, and growth factors (see Fig. 59-5). Of note, RANK is broadly expressed, and these mouse studies indicate that the RANK/RANKL system functions in tissues beyond bone. For instance, RANK/RANKL regulates lymph node formation and lactational mammary gland development in mice.172,177 OPG has nonskeletal functions too, as it protects large arteries of mice from medial calcification.174
Other regulatory molecules have been implicated recently in the late stages of osteoclast differentiation, the fusion process, and activation of resorption. Co-stimulatory molecules acting in concert with M-CSF and RANKL to complete osteoclastogenesis include proteins containing an immunoreceptor tyrosine-based activation motif (ITAM) domain that is critical for the activation of calcium signaling and is found in adapter molecules like DNAX-activating protein (DAP)12 and the Fc receptor γ (FcRγ).178 The resultant increase in intracellular calcium is required for activation of NFATc1. The fusion of mononuclear osteoclast precursor cells into mature multinucleated osteoclasts is regulated by a membrane protein called dendritic cell-specific transmembrane protein (DC-STAMP). DC-STAMP–deficient cells failed to fuse, and these mononuclear osteoclasts had reduced resorptive efficiency in vitro. Consequently, DC-STAMP–deficient mice exhibited increased bone mass.179 These data may suggest that multinucleation and osteoclast enlargement could be associated with a higher degree of resorption efficiency.180,181 Pathologically huge osteoclasts, containing substantially more nuclei than normal osteoclasts do, are seen for instance in Paget’s disease of bone (up to 100 nuclei per cell; normal range is 3 to 20 nuclei per cell), in which localized excessive bone remodeling is initiated by increases in osteoclast-mediated bone resorption.182
Additional studies on the regulatory components of osteoclastogenesis not only will expand our basic understanding of the molecular mechanisms of osteoclast differentiation during bone development and remodeling but also offer opportunities to develop therapeutic means of intervention in osteoclast-related diseases. OPG and soluble recombinant RANK suppress osteoclastogenesis, while antibodies to RANK can stimulate osteoclast formation.183 From the clinical point of view, the RANKL signaling pathway thus holds great promise as a strategy for suppressing excessive osteoclast formation in a variety of bone diseases, including osteoporosis, autoimmune arthritis, periodontitis, Paget’s disease, and bone tumors/metastases.
Bone Resorption: Osteoclast Action and Proteolytic Enzymes
Bone resorption involves both dissolution of bone mineral and degradation of organic bone matrix. Osteoclasts are highly specialized to perform both of these functions. Upon activation of mature multinucleated osteoclasts, the cells attach themselves firmly to the bone surface, using specialized actin-rich podosomes (actin ring), through cytoskeleton reorganization and cellular polarization.184–187 Within these tightly sealed zones of adhesion to the mineralized matrix, the osteoclasts form convoluted, villus-like membranes called “ruffled borders,” which drastically increase the surface area of the cell membrane facing the resorption lacuna (Howship’s lacuna). Via these ruffled membranes, the osteoclasts secrete abundant hydrochloric acid (involving the vacuolar H+-ATPase proton pump), mediating acidification of the compartment between the cell and the bone surface, as well as a myriad of enzymes such as lysosomal cathepsins, the phosphatase TRAP, and proteolytic MMPs (see later). The acidity of the environment leads to dissolution of the mineral phase (crystalline hydroxyapatite), activation of lytic enzymes, and digestion of organic matrix compounds (see Fig. 59-5). The sealing mechanism allows localized dissolving and degrading of the mineralized bone matrix, while simultaneously protecting neighboring cells from harm.188,189 During the resorption process, dissolution of hydroxyapatite releases large amounts of soluble calcium, phosphate, and bicarbonate. Removal of these ions is needed (e.g., to maintain the acidic pH in the resorption lacuna) and apparently involves vesicular pathways and direct ion transport via different ion exchangers, channels, and pumps. The degradation products of the organic matrix after enzymatic digestion are transcytosed through the cell for secretion at the basolateral membrane.188,189
These complex processes of osteoclast recruitment, polarization on the bone surface, and export of acid and enzymes are orchestrated by many factors, including RANKL,190–192 as well as by integrin-mediated signaling from the bone matrix itself.193–195 The latter, which is particularly represented by the αvβ3 integrin in osteoclasts, was suggested to be important for osteoclast functioning based on the finding that inhibition of signaling through this αvβ3 integrin inhibited osteoclast-mediated bone resorption in vitro and in animal models of osteoporosis and malignant osteolysis (reviewed in refs. 196, 197). Integrins are heterodimeric cell-surface receptors, composed of an α and a β subunit, that mediate cell–matrix interactions and thus adhesion. The αvβ3 integrin, among various integrins the most highly expressed in osteoclasts, recognizes RGD(Arg-Gly-Asp)-containing matrix proteins such as vitronectin, osteopontin, and bone sialoprotein. Several components of the αvβ3 integrin signaling pathway localize to the sealing zone of actively resorbing osteoclasts, suggesting that they play a role in linking the matrix adhesion of osteoclasts to cytoskeletal organization, cell polarization, and activation for bone resorption. For these reasons, αvβ3 integrin appeared as a favorable candidate target for antiresorptive therapeutic interventions; in fact, small molecule inhibitors of the integrin are in clinical trial for the treatment of osteoporosis.194,198
Among the molecules that are important for the functional activity of osteoclasts per se, such as β3 integrin, but also c-Src, cathepsin K, carbonic anhydrase II, TRAP, and several ion channel proteins, many have been found to cause an osteopetrotic phenotype when deleted in mice or altered in humans. The absence of these genes does not affect the differentiation into morphologically normal osteoclasts; however, the osteoclasts are not functional, and they fail to resorb bone effectively.81 For instance, cathepsin K, the key enzyme in the digestion of bone matrix by its activity in degrading type I collagen, is highly expressed by activated osteoclasts and secreted in the resorption lacuna.199–201 Its deletion in mice led to osteopetrosis,201–203 and mutations in the human cathepsin K gene cause pycnodysostosis.204 Highly selective and potent cathepsin K inhibitors have been developed recently and have been shown to be useful antiresorptive agents for the treatment of osteoporosis, as well as promising therapeutic tools to reduce breast cancer–induced osteolysis and skeletal tumor burden.205–208
Besides cathepsin K, several proteolytic enzyme groups are involved in the degradation of organic components (collagens and proteoglycans) of bone and cartilage matrices after the mineral is dissolved.209–211 One of these is the MMP family, which constitutes over 25 members, including secreted collagenases, stromelysins, gelatinases, and membrane-type (MT)-MMPs.212,213 MMPs are synthesized as latent proenzymes that, upon proteolytic activation, can degrade numerous extracellular matrix components. As such, they are involved in the development, growth, and repair of tissues, but also in pathologic conditions associated with excessive matrix degradation, such as rheumatoid arthritis, osteoarthritis, and tumor metastasis.210,213–215 Several MMPs, including MMP9 and MMP14 (also known as MT1-MMP), are highly expressed in osteoclasts/chondroclasts,128,216,217 but they are produced by many other cell types as well. Both of these molecules play a role in the cartilage resorption process associated with invasion by osteoclasts during endochondral ossification.122,128,218–220 MMPs and proteolytic enzymes containing a disintegrin and metalloprotease domain (ADAMs) also possibly affect osteoclastogenesis per se, by modulating the bioavailability and presentation of RANKL through the proteolytic cleavage of its transmembrane form to soluble RANKL.221,222
Finally, after a limited period of resorptive activity, the osteoclast is thought to die via apoptosis (see later),223,224 and the resorbed area of cartilage or bone is, in conditions of development, growth, and bone health, efficiently replaced by newly formed bone through the action of osteoblasts.
Osteoblastogenesis
The final step required to rebuild cartilage to bone during development consists of the differentiation of osteoblasts and the deposition of mineralized bone tissue by this specialized cell type. Osteoblasts are mesenchymal cells thought to descend from mesenchymal stem cells that are pluripotent and have the capacity to differentiate into a variety of cell types, including muscle cells (myoblasts), fat cells (adipocytes), chondrocytes, and osteoblasts (Fig. 59-6).225–227 Differentiation of mesenchymal stem cells into distinct lineages is regulated by the expression of different transcription factors. MyoD transcription factors (MyoD, Myf5, and myogenin) regulate myogenic differentiation; C/EBP family (C/EBPβ, C/EBPδ, and C/EBPα) and PPARγ2 transcription factors regulate adipocyte differentiation (see Fig. 59-6).228 In certain settings, osteoblasts and chondrocytes seem to share a common precursor, termed osteochondroprogenitor. Specific transcription factors direct the osteochondroprogenitors into the chondrocyte (the Sox family transcription factors Sox9, Sox5, and Sox6) or the osteoblast lineages (β-catenin, Runx2, and osterix; discussed later) (see Fig. 59-6).31,229,230
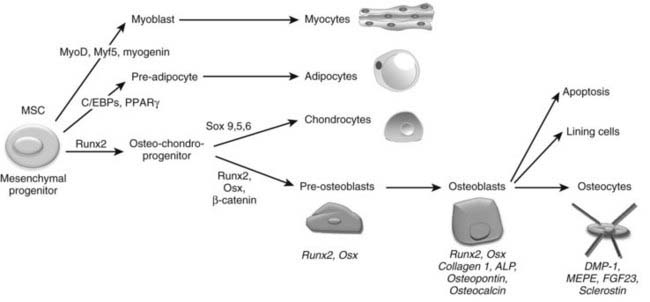
FIGURE 59-6. Osteoblast differentiation. Osteoblasts are derived from mesenchymal stem cells (MSCs) or progenitors that can become myocytes, adipocytes, or chondrocytes (driven by the indicated transcription factors) or can be directed into the osteoblast lineage, possibly through an osteochondroprogenitor intermediate. The transcription factors Runx2, Osx, and β-catenin mediate osteoblast differentiation and functioning. Progressive osteoblast differentiation is characterized by changes in gene expression typifying specific stages as indicated. See text for details.
Committed pre-osteoblasts further differentiate into mature osteoblasts that secrete large amounts of type I collagen and other matrix components, which together are called osteoid. Subsequently, osteoblasts direct the mineralization of the osteoid in a process that requires active alkaline phosphatase (ALP), expressed on the osteoblast membrane. These mature osteoblasts express characteristic genes, including the gene encoding osteocalcin.231 Ultimately, the cells die through apoptosis, or are converted to bone-lining cells, or become embedded within the bone matrix as osteocytes. Osteocytes are found dispersed throughout the bone matrix and have the potential to live as long as the organism itself. In fact, osteocytes are the most abundant cell type in bone, constituting over 90% of adult bone cells.232,233 As osteoblasts become encased by the mineralized bone matrix that they themselves synthesized, they dramatically change their shape, sending out numerous dendritic processes that run inside lacunar cannaliculi.234,235 These processes allow osteocytes to communicate with each other, with the vasculature, and with cells on the bone surface.233,236–239 Osteocytes are well positioned to sense and respond to mechanical forces, and a growing body of evidence documents that osteocytes mediate the actions of mechanical forces to increase bone mass (see later). Osteocytes synthesize a series of proteins unique to this most differentiated member of the osteoblast lineage. These include dentin matrix protein (DMP)-1, matrix extracellular phosphoglycoprotein (MEPE) and FGF23, secreted proteins involved in systemic phosphate homeostasis, and sclerostin (SOST), an inhibitor of Wnt action (see later).237,240–244 Despite their relative inactivity compared with osteoblasts, osteocytes play a central role in the determination and maintenance of bone structure (see later).
A number of crucial transcription factors and signaling pathways determining osteoblastogenesis have been identified, and at least some of those are currently known to play a role in inherited human bone disease. Here, we will focus on discussing Wnt/β-catenin signaling and the transcription factors Runx2 and Osx, and we will briefly touch on other important signals, including BMPs, hedgehogs (Hh), and FGFs.
Wnt/β-Catenin Signaling
Wnts are a large family of secreted growth factors (19 different members in mouse and human genomes) that play essential roles in multiple developmental processes. Wnts are also required for adult tissue maintenance, and perturbations in Wnt signaling can lead to tumor formation and other diseases.17,245–248 In the skeletal system, mutations in Wnt signaling components lead to skeletal malformations and diseases such as osteoporosis-pseudoglioma249 and osteoarthritis.250
Wnts can transduce their signals through several different downstream signaling pathways. The best understood pathway is the canonical or Wnt/β-catenin pathway (Fig. 59-7).251 Central to this pathway is the regulation of the protein stability of β-catenin, which acts as a transcription factor in the canonical Wnt pathway and is also involved in cell adhesion by binding to cadherins. In the absence of Wnts, cytoplasmic β-catenin is constitutively degraded through its phosphorylation by glycogen synthase kinase 3-β (GSK3-β) in a large protein complex brought together by axin and adenomatous polyposis coli (APC).245,247,252,253 Phosphorylated β-catenin is recognized by a β-transducin repeat containing protein (β-TrCP) that targets it for proteasome-mediated degradation. Upon Wnt stimulation, the ligands bind to two synergistically acting families of Wnt (co)receptors, the Frizzled (Fz) receptor family members and low-density lipoprotein receptor-related proteins (LRP5 or LRP6), leading to the recruitment of axin to LRP5/6 in the plasma membrane. The sequestering of axin at the plasma membrane likely leads to disassembly of the β-catenin destruction complex, thus inhibiting the phosphorylation/degradation of β-catenin.254–259 Stabilized β-catenin protein then accumulates in the cytoplasm and translocates to the nucleus, where it interacts with members of the T cell factor/lymphoid enhancer factor (TCF/LEF) family of DNA-binding transcription factors to regulate the expression of downstream target genes (see Fig. 59-7).260–262
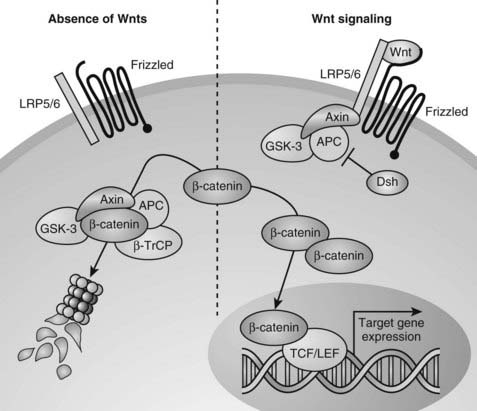
FIGURE 59-7. Canonical Wnt/β-catenin signaling pathway. In the absence of Wnt signals (left), β-catenin is associated with glycogen synthase kinase 3-β (GSK3-β) in a destruction complex that also contains Axin and adenomatous polyposis coli (APC). A hyperphosphorylated β-catenin is created by GSK3β and is bound by the β-transducin repeat containing protein (β-TrCP) component of the ubiquitin ligase complex, targeting β-catenin for proteasomal degradation. Wnt signaling through the Frizzled and LRP5/6 receptor complex (right) leads to inhibition of GSK3β through activation of Dishevelled (Dsh). Consequently, when Wnt signaling is active, β-catenin is stabilized, and the level of β-catenin in the cytoplasm becomes elevated. Accumulated β-catenin can translocate to the nucleus to interact with T cell factor/lymphoid enhancer factor (TCF/LEF) proteins and mediate transcription of target genes.
During the past decade, canonical Wnt signaling has been shown to play a significant role in the control of osteoblastogenesis and bone formation. Recent genetic studies analyzing conditional β-catenin loss- and gain-of-function mouse models provided compelling evidence that β-catenin is a crucial transcription factor determining the osteoblast lineage commitment of early osteochondroprogenitors, by inducing osteoblastic and suppressing chondrocytic differentiation.17,263 Indeed, inactivation of β-catenin in mesenchymal progenitor cells blocked osteoblast differentiation, and mesenchymal cells in the perichondrium and calvarium differentiated into chondrocytes instead.16,18,264 In addition, recent evidence showed that β-catenin plays an important role in the coupling between osteoblast and osteoclast activity by stimulating differentiated osteoblasts to produce OPG, an inhibitor of osteoclast formation (see earlier).265,266
In several clinical cases, mutations have been found in the Wnt receptor complexes that are associated with changes in bone mineral density and fractures. Loss-of-function mutations in LRP5 receptors cause osteoporosis-pseudoglioma syndrome,249 whereas gain-of-function mutations in the same group that render LRP5 with reduced affinities for DKK1 and sclerostin (SOST), secreted antagonists of canonical Wnt signaling, result in high bone mass phenotypes.267–271 These actions of LRP5 are indirect actions on bone: Activation of LRP5 in the duodenum suppresses serotonin secretion, and the lower blood levels of serotonin lead to an increase in bone mass through less activation of serotonin Htr1b receptors on osteoblasts.272
The protein inhibitors of Wnt signaling may have additional clinical relevance. For example, osteolytic bone lesions in patients with multiple myeloma are associated with the production of DKK1,273,274 and homozygous loss-of-function mutations in SOST have been identified in patients diagnosed with sclerosteosis, a disfiguring disease associated with high bone mass.275,276 Mutations affecting regulation of SOST transcription also cause van Buchem disease, a disease that resembles sclerosteosis.241,277 These findings highlight the important role of canonical Wnt signaling in regulating human bone mass. Because osteocytes are the major producer of SOST, inhibition of this Wnt antagonist may offer a promising strategy for preventing bone loss.17,278,279 Extensive research therefore is being conducted in this area based on the prospect that it may lead to the identification of targets of pharmacologic intervention useful in the management of osteoporosis.
Runx2 and Osx
Runx2, runt-related transcription factor 2 (previously known as core-binding factor a1 [Cbfa1], Osf2, or AML3), a transcription factor of the ancient runt family, has been demonstrated as absolutely essential for the induction of osteoblast differentiation and endochondral and intramembranous bone formation. Runx2-deficient mice completely lack osteoblasts and do not form bone at all; instead a completely cartilaginous skeleton develops without any true bone matrix.280,281 In heterozygous (haploinsufficient) Runx2 mutant mice, the defect in osteoblast differentiation is limited to intramembranous bones.280 The resulting phenotype in these mice closely resembles the cleidocranial dysplasia (CCD) syndrome in humans, a dominantly inherited developmental disorder of bone, and RUNX2 was found to be mutated in most CCD patients.282,283 Consistent with its function as an early transcriptional regulator of osteoblast differentiation, Runx2 is an early molecular marker of the osteoblast lineage, being highly expressed in perichondrial mesenchyme and in all osteoblasts.280,284 Hypertrophic chondrocytes also express Runx2, and Runx2 plays important roles in cartilage biology, as was mentioned earlier.
Runx2 can be sufficient to induce osteoblast differentiation in vitro.285 Moreover, Runx2-null calvarial cells spontaneously differentiate into adipocytes and differentiate into chondrocytes in the presence of BMP-2 in vitro, but they do not differentiate into osteoblasts.286 Thus, Runx2 is both sufficient and essential for differentiation of mesenchymal cells into osteoblasts, and it inhibits their differentiation into adipocytes and chondrocytes. Runx2 mediates osteoblast differentiation by inducing ALP activity, by regulating the expression of a variety of bone matrix protein genes, and by stimulating mineralization in immature mesenchymal cells and osteoblastic cells.287 Furthermore, Runx2 regulates the expression of RANKL and OPG in osteoblasts, thus affecting osteoclast differentiation (see earlier).288,289
The DNA-binding sites of Runx2 have been identified in major osteoblast-specific genes, including the genes that encode type I collagen, (Col1a1), osteopontin, osteonectin, bone sialoprotein, osteocalcin, and Runx2 itself, and Runx2 induced the expression of these genes or activated their promoters in vitro.287 It is puzzling that although Runx2 strongly induced osteocalcin expression in vitro,285,290 its overexpression in osteoblasts in vivo severely reduced osteocalcin expression.291 Yet, expression of a dominant-negative form of Runx2 in osteoblasts also led to virtual absence of osteocalcin expression and caused impaired postnatal bone formation.292 What is clear from these and other findings is that the regulation of different stages of osteoblast differentiation by Runx2 is very complex; Runx2 transcriptional regulation involves interactions with a myriad of transcriptional activators and repressors and other co-regulatory proteins that are under continued investigation. The current model is that Runx2 triggers the expression of major bone matrix protein genes and the acquisition of an osteoblastic phenotype at an early stage of osteoblast differentiation, while inhibiting the late osteoblast maturation stages and the transition into osteocytes. As such, Runx2 may play an important role in maintaining a supply of immature osteoblasts.287
Runx2 also regulates the expression of Osterix (encoded by the Osx or Sp7 gene), an SP family transcription factor with three zinc-finger motifs. Osterix is expressed in osteoblast progenitors, in osteoblasts, and at a lower level in (pre-)hypertrophic chondrocytes.293 Similar to Runx2-deficient mice, mice lacking Osterix showed complete lack of osteoblasts and absence of both intramembranous and endochondral bone formation.293 Thus, Osterix is a third transcription factor that is essential for osteoblast differentiation. Because Runx2 is expressed in the mesenchymal cells of Osx-null mice but Osterix is not expressed in Runx2-null mice, it can be concluded that Osterix acts downstream of Runx2.293 Furthermore, the Osx gene contains a consensus Runx2-binding site in its promoter region, suggesting that Osterix might be a direct target of Runx2.294 The transcriptional activity of Osterix involves its interaction with NFATc1, cooperatively forming a complex that binds to DNA and induces the expression of Col1a1.295 The subtleties of how Osterix regulates osteoblast differentiation and function and which osteoblastic genes are directly regulated by Osterix have not yet been elucidated. Expression of genes characteristic of mature osteoblasts (such as those encoding bone sialoprotein, osteopontin, osteonectin, and osteocalcin) was absent in cells surrounding chondrocytes in Osx-null mice, and instead these cells express genes characteristic of chondrocytes (Sox9, Sox5, Col2a1).293 Osterix has also been reported to inhibit chondrogenesis in vitro.296,297 Thus, Osterix may be important for directing precursor cells away from the chondrocyte lineage and toward the osteoblast lineage. Overall, it is currently thought that Runx2 has a crucial role in the earliest determination stage of the osteoblast lineage, driving mesenchymal progenitors to become pre-osteoblasts, while Osterix regulates at a later stage the differentiation of pre-osteoblasts to functional, bone-forming osteoblasts expressing high levels of osteoblast markers (see Fig. 59-6).
Other Factors and Signaling Molecules Involved in Osteoblastogenesis
Besides β-catenin, Runx2, and Osterix, various other (non–bone-specific) transcription factors are involved in osteoblast differentiation and function, albeit not to a similar critical extent, as their inactivation does not completely abrogate bone formation. These include ATF4,298–300 Msx1 and Msx2,301–304 Dlx5, Dlx6, and Dlx3,305–308 Twist,309,310 activator protein-1 (AP1) and its related molecules (Fos/Jun),311–315 and Schnurri-2 and -3.230,316–322 A myriad of morphogens and signaling molecules control the activity of the transcription factors just described. These molecules include Wnts, as discussed before, as well as locally produced BMPs and TGFβ, Hedgehogs (particularly Ihh), FGFs, Notch ligands, IGFs, PDGF, and systemic factors like PTH, GH, prostaglandins, estrogens, androgens, 1,25(OH)2D3 and glucocorticoids.81,97,323
Signaling pathways involving BMPs, Ihh, and FGFs have been mentioned previously in this chapter for their roles in chondrogenesis, but these signals also exert important functions in osteoblastogenesis and bone formation. Among BMP family members, BMP-2, BMP-4, and BMP-7 have been studied most extensively for their roles in osteogenesis during development, postnatal bone formation and remodeling, and fracture repair.15,40,49 The double knockout of BMP-2 and BMP-4 in the limb completely disrupted osteoblast differentiation, demonstrating the crucial roles of these two BMPs in osteoblast differentiation.49 Effects of BMP signaling in later stages of osteoblast differentiation are suggested by studies using BMP antagonists. Targeting of noggin overexpression to differentiated osteoblasts by the osteocalcin promoter results in osteopenia by 8 months of age.324 Likewise, overexpression of gremlin, another BMP antagonist, in differentiated osteoblasts results in reduced bone mineral density and fractures.325
BMP-2 has been shown to play a critical role in osteogenic differentiation: It promotes the commitment of pluripotent mesenchymal cells to the osteoblast lineage326–329 and has been demonstrated to induce the expression of both Runx2 and Osx during osteoblastogenesis.327,330–333 The precise mechanisms responsible for BMP-2-mediated gene regulation during osteoblast differentiation and bone formation are not well elucidated because of the considerable complexity and involvement of a myriad of interacting pathways. Nevertheless, the activity of BMP-2 as a potent inducer of bone formation is being applied to repair bone defects in humans.323,334,335 A recent human genetic study indicated that polymorphisms in the BMP-2 gene are linked to a high risk for osteoporosis.336
The Hedgehog family member Ihh is required for endochondral but not for intramembranous bone formation, by controlling osteoblast differentiation in the perichondrium of long bones. Ihh-null mice completely lack endochondral ossification because of the lack of osteoblasts.72,337 In these mice, Runx2 is expressed in chondrocytes but not in perichondrial cells. As well, nuclear β-catenin is absent in the perichondrial cells of Ihh-deficient mice.16 Thus, Ihh is required for inducing the initial activity of Runx2 and β-catenin in perichondrial cells and triggering them to become endochondral osteoblasts, thereby coupling chondrocyte maturation with osteoblast differentiation during endochondral ossification. Chondrocyte-derived Ihh remains crucial for sustaining trabecular bone in the postnatal skeleton.80 Skeletal abnormalities have been described in mutant mice lacking some of the intracellular mediators of Ihh signaling termed Gli proteins (three related transcription factors Gli1, Gli2, and Gli3)338–341; Gli2 mediates Ihh-induced osteoblast differentiation in mesenchymal cell lines by associating with Runx2 and stimulating its expression and function,342 as well as by inducing BMP-2 expression.343
FGF signaling has been implicated in the proliferation of immature osteoblasts and the anabolic function of mature osteoblasts in vivo.50–52,344–348 Whether and how osteoblast differentiation per se is affected by FGFs is currently elusive, but recent evidence indicates that FGF signaling induces BMP-2 expression and stimulates the expression and transcriptional activities of Runx2.349–354 Conversely, Runx2 has been demonstrated to form a complex with Lef1 or TCF that binds to the promoter region of the gene encoding FGF18, inducing its expression.355 These findings underscore once more how the various pathways that are essential for endochondral and intramembranous bone development physically and functionally converge. Moreover, the ultimate outcome relies on the complex integration of stimulatory and inhibitory signaling. For instance, Notch signaling suppresses osteoblast differentiation by diminishing Runx2 transcriptional activity via the Wnt/β-catenin pathway, actions that may be required to maintain a pool of undifferentiated mesenchymal progenitors in the bone marrow.356–359
Further characterization of the genetic pathways regulating osteoblastogenesis is under way and has been boosted by high hopes of finding effective therapeutic strategies to treat skeletal diseases and prevent pathologic bone loss, as in osteoporosis. Indeed, in this ever more widespread condition, current treatments as yet still most often target osteoclastic bone resorption, while compounds directed at the stimulation of osteoblastic bone formation are much sought after (see later).
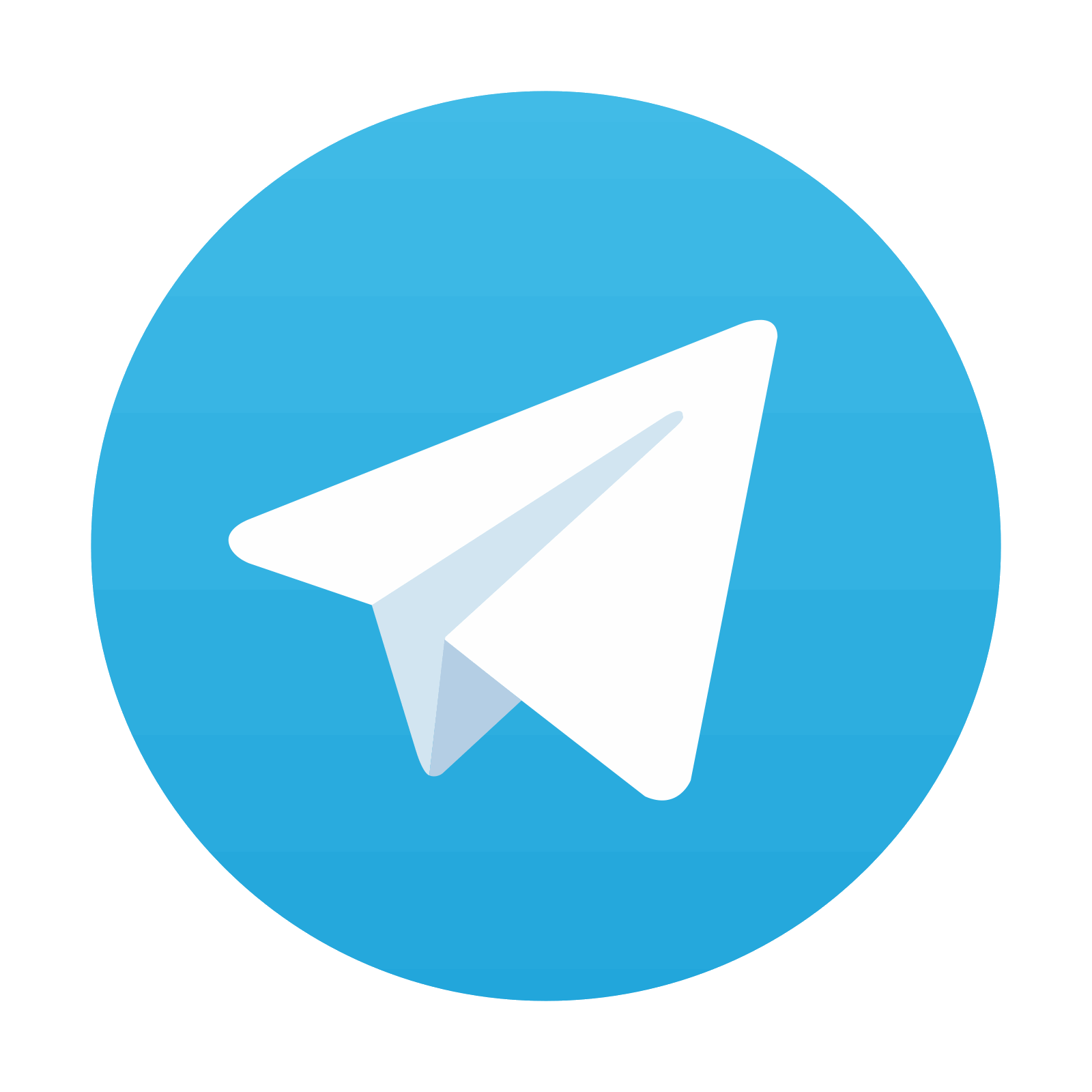
Stay updated, free articles. Join our Telegram channel
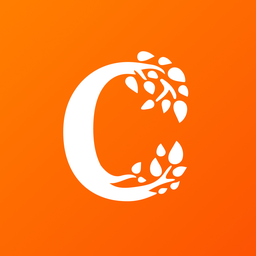
Full access? Get Clinical Tree
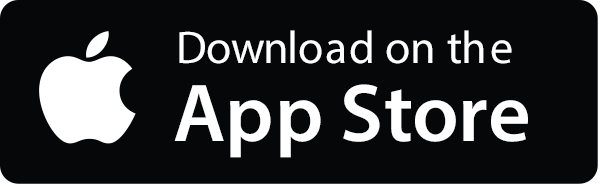
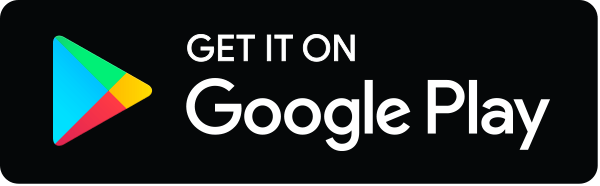