Fig. 13.1
(a) There is a symbiotic relationship between the microbiome and the host, as the host provides a habitat and resources including nutrients for the microbiome. In turn, the microbiome aids in the healthy development and functioning of animal tissues such as the immune system, GI tract, and nervous system. (b) The host and the microbiome communicate (interkingdom signaling) through catecholamines and neurotransmitters (such as GABA). The host’s catecholamine production changes the proliferation of bacteria and in turn bacteria produce neurotransmitters (GABA) that alter the hosts’ behavior
The holobiont paradigm is represented, to some extent, by the hygiene hypothesis (originally conceived by Strachan in 1989). This hypothesis proposes that human cells have co-evolved with pathogenic microorganisms (including infectious bacteria, human viruses, helminthes, etc., see Fig. 13.2). According to this hypothesis the interactions between human cells and pathogens are essential for a healthy immune system development. This implies that abnormalities in this interaction (such as excessive hygiene and lack of infections) in early life might be partially responsible for the development of immunologic diseases such as asthma, allergies and other inflammatory conditions later in life (Penders et al. 2007; Rook 2009; Sommer and Bäckhed 2013). This may explain the lower rates of allergic diseases in people from developing countries compared with industrialized ones. Research findings on the association of the microbiome and asthma complement the hygiene hypothesis, as both support the role of symbiotic (commensal and pathogenic) organisms on asthma development and exacerbation (Azad et al. 2013; Bendiks and Kopp 2013; Daley 2014).
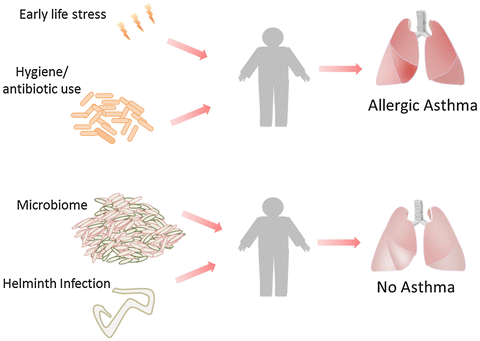
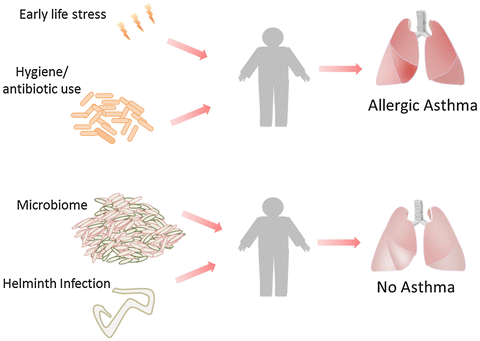
Fig. 13.2
Asthma development has been linked to early life stress and antibiotic treatment. Certain parasite infections (such as helminth infections) and the microbiome can provide protection against asthma
Recent studies suggest that the composition of the microbiome might impact the development of the human stress systems including the hypothalamus–pituitary–adrenal axis (HPA axis; Sudo et al. 2004; Sudo 2012) and the adrenergic system (Goehler et al. 2005). This co-evolution has left signatures in microorganism genomes as evidence suggests that microbial pathogens can react to human stress hormones and thereby become more virulent and increase their growth rate (Bansal et al. 2007; Marks et al. 2013; Gonzales et al. 2013; Dickson et al. 2014). It is also possible that human hormones may alter the commensal members of the microbiome (Belay and Sonnenfeld 2002; Freestone et al. 2012).
In this chapter we will explore interactions between psychological factors (anxiety, depression, and stress) and the composition of the microbiome and their links to development and exacerbation of asthma and allergies. We will begin by reviewing the relation between psychological factors and atopic diseases such as asthma. This includes the exploration of how early life stress might be involved in the development of psychological and inflammatory conditions, specifically allergic asthma. The effect of microbial colonization during early years of life on asthma and HPA axis development will then be discussed. Following this we will consider some of the evidence for stress-induced alterations in bacterial behavior and will discuss the impact that this might have on asthma exacerbation. Finally, we assess future research directions and clinical implications involving the role of bacterial colonization, with potential avenues for novel interventions to ameliorate inflammatory and allergic conditions as well as psychiatric disorders.
13.2 Asthma
Asthma is a chronic disease of the airways. It is characterized by chronic inflammation, airway obstruction, airway remodeling, and increased mucus production, which result in intermittent symptoms of coughing, shortness of breath, wheezing, and chest tightness that vary in intensity across time (National Heart, Lung, and Blood Institute/National Asthma Education and Prevention Program [NHLBI/NAEPP], 2007). Approximately 300 million individuals suffer from asthma worldwide, making it one of the most prevalent chronic diseases among adults and the most common chronic diseases in children (Masoli et al. 2004). Asthma accounts for 1 % of disability-adjusted life years (DALYs) lost and its overall burden amounts to a loss of approximately 15 million DALYs per year (Bousquet et al. 2005).
The pathogenesis of asthma is complex because the disease can have a variety of underlying immune processes depending on the subtype (endotypes, Lötvall et al. 2011; Holgate 2002; Wenzel 2006). Atopic or allergic asthma has been characterized by elevations in type 2 T helper (Th2) cells (and Th2 cytokines IL-4, IL-5, IL13) relative to type 1 T helper (Th1) cells (and Th1 cytokines IL-2, IFN-γ). Th2 cells are involved in allergic processes and result in an excess of the antibody immunoglobulin E (IgE; Lötvall et al. 2011). Atopy prevalence can vary depending on the demographics including age, gender, and race. In the United States atopy accounts for 56.3 % of asthma cases in individuals that are 6–59 years old (Arbes et al. 2007). For young men with higher education levels living in metropolitan areas the prevalence of atopy can be as high as 74.1 %. Other asthma subtypes are characterized by inflammation that is caused by other immune cells such as natural killer (NK) cells, neutrophils, macrophages (Matangkasombut et al. 2009), bronchial epithelial cells, or dendritic cells (Lambrecht and Hammad 2003). For example, in a particular subtype, overactivity of NK cells can exacerbate allergic processes by producing Th2 cytokines. NK cells can also foment non-allergic asthma by causing airway hyperreactivity through reduction of regulatory T (T reg) cells and an increase in neutrophil recruitment by way of IL-17 production (Matangkasombut et al. 2009). Other asthma subtypes are non-eosinophilic and are characterized by Th1 over expression (Zhu et al. 2010). This review will focus mostly on atopic asthma over other subtypes.
There is a high prevalence of psychological disorders among patients with asthma. Associations have been found between asthma and anxiety symptoms (Slattery and Essex 2011) and several anxiety disorders (Goodwin et al. 2004; Lavoie et al. 2011). Depression prevalence also appears to be higher among asthma patients (Slattery and Essex 2011; Wong et al. 2013), although the literature is more variable (Opolski and Wilson 2005). Negative emotions or mood have been linked to reduced airway function (Ritz et al. 2013) or worsening of asthmatic symptoms (Rietveld et al. 1999), as have depression (Richardson et al. 2006), adverse life events (Sandberg et al. 2000), low socioeconomic status, and stress (Chen et al. 2010).
Stress-induced changes in immune function have been proposed as important mechanisms that could explain asthma symptom worsening in the context of psychosocial stress (Kang et al. 1997; Liu et al. 2002; Chen et al. 2010; Trueba and Ritz 2013). A substantial number of studies suggest that the association between inflammation and psychopathology can start early in life. Studies have examined the impact of adversity and mental health in childhood on subsequent allergic condition development. Epidemiological studies have shown an association between a history of asthma and anxiety disorders in children (Ortega et al. 2002) and adolescents (Slattery and Essex 2011). Prospective longitudinal research has shown a relationship between early life adversity or young adulthood anxiety disorder and the subsequent the development of asthma (Hasler et al. 2005; Scott et al. 2008) Research also suggests that maternal stress in early life (from birth until age 7) is associated to increased likelihood of childhood asthma (Kozyrskyj et al. 2008). Although there are several proposed immune mechanisms, much remains to be learned about the pathways underlying the association between life stress and subsequent asthma development.
It has been suggested that the effect of stress on immune processes can start as early as in the intrauterine phase (Wright 2010; Wright et al. 2010). Indeed, studies have found that maternal psychological stress during gestation was associated with greater likelihood of asthma in childhood (Cookson et al. 2009). High levels of umbilical cord immunoglobulin E (cIgE; an allergy-related immunoglobulin) have been associated with family and maternal history of atopic conditions and studies have found that psychosocial factors such as stress are related to elevated cIgE levels (Lin et al. 2004). Similarly, the Urban Environment and Childhood Asthma Study examined 557 families and found that prenatal maternal stress (e.g. violence, financial difficulties) was related to an increase in a asthma-related Th2 cytokine (IL-13) release by cord blood mononuclear cell (CBMC) (Wright et al. 2010). This finding suggests that early stress heightens asthmatic immune responses in perinatal infants and this could prime infants to develop asthma (Wright 2010).
13.3 Asthma and the HPA Axis
A characteristic that asthmatics and individuals with psychopathology seem to have in common are abnormalities in their physiological response to stressors. There is evidence to suggest that stress system malfunctioning is related to the development of asthma (Buske-Kirschbaum et al. 2003; Buske‐Kirschbaum and Hellhammer 2003; Wright 2007; Priftis et al. 2008) and psychopathology (Glover et al. 2010; Glover 2011).
The HPA axis is one of two important systems that modulate physiological responses to stress. The end result of the HPA axis activation in response to stress is the release of adrenocorticotrophic hormone (ACTH) from the pituitary gland (Webster et al. 1998) and glucocorticoids from the adrenal cortex (Elenkov and Chrousos 1999). The other stress system is the locus ceruleus-norepinephrine (LC-NE)/sympathetic nervous system, which mediates the fight-or-flight response. Activation of the sympathetic nervous system releases norepinephrine from presynaptic terminals at organs and glands. In addition, the adrenal medulla releases catecholamines into the blood stream when stimulated by sympathetic nerve terminals (Elenkov et al. 2000).
Previous research has found an association between HPA axis dysregulation and allergic asthma. One study observed that children with allergic asthma compared to controls had a blunted cortisol response when exposed to standard laboratory stressor (free speech and mental arithmetics under evaluative threat). None of these children were on corticosteroid medications (Buske-Kirschbaum et al. 2003). Similarly, children with allergic dermititis also have showed blunted cortisol responses to this type of laboratory stressor (Buske-Kirschbaum et al. 1997) and these same findings have been replicated in adolescents with atopic disorders (Wamboldt et al. 2003) and adults with asthma (Buske-Kirschbaum et al. 2002). There is also evidence that the circadian rhythm of cortisol in infants at risk for allergic diseases (defined by having parents with allergic disease) is flattened such that they have a reduced morning cortisol response and lower diurnal cortisol release (Ball et al. 2006). Studies have found that this altered HPA axis response is independent of corticosteroid use. Indeed, there is some evidence that use of cortisosteriods could an actually reverse the adrenal suppression that observed in asthmatics display (Priftis et al. 2006, 2008). In drawing conclusions from these findings it is important to consider the complexity of asthma with different subtypes (endotypes, see above) each of which involve distinct immune processes. Most of the literature in this area has focused on dysregulation of the HPA axis and on atopic conditions, which means that findings might not generalize to all asthma subtypes.
One potential mechanism that could explain the altered HPA axis responses in asthma and atopy is an elevation in circulating inflammatory cytokines. Elevations in interleukin 1 (IL-1) can lead to the release of glucocorticoids such as cortisol, as IL-1 stimulates corticotropin-releasing hormone (CRH) release which initiates a cascade of HPA axis activation (Glaser and Kiecolt-Glaser 2005). This relationship is bidirectional, such that hyperactivity of the HPA axis can lead to glucocorticoid resistance, which can result in exaggerated inflammatory processes (Raison et al. 2006) and a shift towards Th2 immune responses (Van Lieshout et al. 2009), which results in asthma exacerbation.
13.4 HPA Axis and Psychopathology
The role of early life stress in the development of both allergic conditions and psychopathology can be attributed in part to alterations in the development of the HPA axis. There is evidence to suggest that there is a critical period of HPA axis development. Abnormalities in the development of the HPA axis during this period might result in permeant dysregulation associated with the development of anxiety symptoms, conduct disorder, or attention deficit hyperactive disorder (ADHD) in adult age (Glover 2011). In a substantial portion of cases of clinical depression the HPA is hyperactive, in that about half of the patients with major depression have heightened cortisol levels (Pariante and Lightman 2008),increased CRH levels in the cerebrospinal fluid, and elevations in CRH messenger RNA and protein in structures of the limbic region (Forsythe et al. 2010). The same study found that about half of depressed patients do not show the normal drop in cortisol levels after being administered dexamethasone, a challenge that typically results in a suppression of cortisol release because dexamethasone is a negative feedback signal for the pituitary gland that should result in a decreased secretion of ACTH and cortisol. Patients also did not respond to the dexamethasone-CRH test (which is more sensitive for major depression than dexamethasone alone), in which dexamethasone is administered at night and CRH injected on the subsequent day in-between cortisol and ACTH measurements (Heuser et al. 1994). Both findings suggested that the negative feedback by glucocorticoids is blocked. This may be caused by a reduction in the number and sensitivity of glucocorticoid receptors expressed by organs and immune cells including those involved in asthma (Pariante and Lightman 2008).
13.5 Intestinal and Airway Microbiomes in Asthmatics
The microbiome residing in the gut and the respiratory tract of asthmatics is distinct from that of healthy individuals. There is some evidence to suggest that infants who develop allergies have a different bacterial composition in their gut than those that are not allergic (Kirjavainen et al. 2001; Björkstén et al. 2001; Ouwehand et al. 2001). Studies have also shown that individuals with asthma have a distinct microbiome in their respiratory tract compared to that of healthy individuals (Hilty et al. 2010; Huang et al. 2011). Using 16S-rRNA genes to determine the bacteria that reside in the airways, Hilty et al. (2010) found larger proportions of Proteobacteria, a phylum that contains potential pathogens such as Haemophilus, Moraxella and Neisseria spp., in the airways of asthmatics in comparison with healthy individuals. Yet another study found that asthmatic airways have a greater diversity of bacterial species. Furthermore, certain bacterial families such as Sphingomonadaceae or Oxalobacteraceae that reside in the airways of asthmatics have been associated with airway hyperresponsiveness (Huang et al. 2011). Taken together, this research suggests that asthma pathogenesis and/or exacerbation might be affected by microbial composition of airway microbiome. However, cause and effect have not been established and there is also the alternative possibility that chronic inflammation of airway mucosa changes the microbiome (Sekirov and Finlay 2009).
13.6 Microbiome, Pathogens and Asthma
Some studies (including meta-analyses) support the hygiene hypotheses as they suggest that the lack of particular infections in early life may be linked to higher predisposition to asthma (Leonardi-Bee et al. 2006; Penders et al. 2007). The nature of the protective mechanism conveyed by infections are not fully understood, as they may constitute direct interactions of the pathogens with human cells, or involve indirect pathways though intestinal infections that also cause changes in the microbiome (Sekirov and Finlay 2009). Asthma and other atopic conditions are more prevalent in developed countries than in developing countries which often differ in the extent to which they emphasise hygiene (Wills-Karp et al. 2001). Another relevant study found that children who went to day care and lived in households with older siblings suffering from recurrent infections were less likely to develop atopic conditions (Ball et al. 2000). In a more recent study, Lynch and colleagues (2014) found that lack of or rare occurrence of exposure to bacteria and allergens in the first 3 years of life made children more likely to develop atopic conditions and wheezing. The main evidence that some microbes can reduce the possibility of developing asthma is associated with research on the hygiene hypothesis. Some examples are infections caused by hookworms (Leonardi-Bee et al. 2006) Toxoplasma gondii, Helicobacter pylori, Mycobacteria tuberculosis, hepatitis A that have been associated with reduced likelihood of developing asthma or allergies (Umetsu et al. 2002). The immune mechanism proposed to underlie the hygiene hypothesis is mostly centered around the effect of microorganisms on the Th1/Th2 balance. Among atopic asthmatics there is imbalance between Th1 and Th2 cells, in that there is an increase in Th2 cells that leads to allergic inflammation in the airways (Mosmann and Coffman 1989). Infections caused by specific pathogens and colonization of certain bacterial species may help balance Th1 and Th2 cell proliferation, by increasing the production T regulatory cells (Treg cells), which protects against atopic asthma development by preventing overproduction of Th2 cells (Umetsu et al. 2002; Macpherson and Harris 2004; Rook and Lowry 2008). However, new perspectives propose that specific microorganisms may have an important role in the maturation of the mucosal and innate immune system. Some bacteria can lead to mucus production (induced by IL-13) as well as induce an increase in IgA levels, both of which protect and strengthen the epithelial barrier (Cookson 2004). It has been proposed that the maturation of the immune system induced by infection is a process that occurs in early life, but findings suggest that some infections continue to be immunomodulary in adults, by causing and mucosal immune “tolerance” towards antigens (Umetsu et al. 2002). This perspective on the hygiene-asthma relationship involving maturation of mucosal and innate immune systems pertains more to development of other asthma subtypes that are not associated with Th2 overexpression but with overexpression of NK cells, neutrophils, or macrophages (Matangkasombut et al. 2009).
The intestinal microbiome has also been linked to asthma. There is some evidence to suggest that infants that who develop allergic asthma have different bacterial composition in their gut than those that are not allergic (Ouwehand et al. 2001; Kirjavainen et al. 2001; Björkstén et al. 2001). The microbiome and the immune system have a complex relationship which may be central to the understanding of asthma pathogenesis. As mentioned above there are many bacterial species including pathogens that colonize and infect the human intestines that have an important role in the healthy development and function of the human immune system (Hooper and Gordon 2001; Sommer and Bäckhed 2013; Round and Mazmanian 2009).
Microbiome disturbances caused by antibiotic treatment during first year of life may predispose to asthma (Droste et al. 2000; Murk et al. 2011). Furthermore, infants born by caesarean section and thereby not exposed to the mother’s gut microbiome) are 20 % more likely to develop asthma (Thavagnanam et al. 2008). The specific intestinal microbiome composition that is related to allergic asthma has not been well defined. Several studies suggest that the Bifidobacteria have a significant role in the development of atopic/allergic conditions. More specifically, infants with allergies tend to have reduced concentrations of Bifidobacterium bifidum (Ouwehand et al. 2001; Umetsu et al. 2002). Similarly, there is evidence that infants with atopic eczema have lower levels of Bifidobacteria and Gram positive organisms (Kirjavainen et al. 2001). It appears that this specific intestinal microbiome profile is associated with allergic conditions regardless of the country of origin. One study found that infants from Estonia and Sweden who developed allergies by the age of 2 had lower concentrations of bifidobacteria and enterococci in their first year and higher levels of clostridia at 3 months and S. aureus at 6 months (Björkstén et al. 2001).
Some findings caution interpretations drawn from experiments of the microbiome and asthma (as is valid for other diseases). Contradictory findings on beneficial versus detrimental effects of infections make it especially challenging to ascertain which bacteria specifically increase the likelihood of developing asthma and which ones decrease it. It seems that several factors might determine if the colonization or infections from a particular bacterial strain is protective or harmful. This includes the age of the host at the time of contact with the bacteria and the amount of bacterial proteins and endotoxin produced by the bacteria (LPS specifically; Zhu et al. 2010). LPS has been shown to have protective effects if exposure is early in life but detrimental if the host is older. LPS is produced by several gram-negative bacteria (e.g., E. coli bacteria), some of which are associated with protective effects such as Helicobacter pylori (Umetsu et al. 2002). Research suggests a time- and dose-dependent relationship: excessive LPS exposure may lead to increased allergen sensitization (Schröder 2009) but early life exposure to LPS protects against atopy (Zhu et al. 2010). Furthermore there is significant variability in the genomes of bacteria. Two bacteria that are of the same species can have very different genes, which ultimately can change the specific interaction that these bacteria have with human cells. These genomic differences could also explain some of the contradictions about the protective versus detrimental effects of bacteria on asthma (Gogarten et al. 2002).
Studies suggest that certain bacterial pathogens that colonize throats of young infants can lead to wheezing, these include Streptococcus pneumoniae, Haemophilus influenzae, Moraxella catarrhalis (Bisgaard et al. 2007) and Chlamydia pneumoniae (Korppi 2010). One study infected mice with Chlamydia pneumoniae subsequently exposed to human serum albumin (HSA) and the infection triggered increased sensitization toward HSA (Schröder et al. 2008).
Some microorganisms promote the mucosal immunity by stimulating mucosal lymphocytes to produce IgA (Cookson 2004), but colonization or infection by other bacteria can actually degrade IgA (Kilian et al. 1995). Certain bacterial species colonizing the respiratory tract produce specific proteases that cleave IgA. These include: Streptococcus mitis biovar 1, Haemophilus influenzae, Haemophilus parahaemolyticus, Streptococcus pneumoniae, and Neisseria meningitidis. Cleavage of IgA is more prevalent in children with a history of atopic conditions than in non-atopic children. This may suggest that IgA1 cleavage by bacterial proteases can contribute to the development of immunological dysfunctions in the mucosa (Kuklinska and Kilian 1984) related to atopy. One study found evidence of elevated levels of IgA1 protease-producing bacteria in the oropharynx of 18-month-old atopic infants specifically Streptococcus mitis biovar 1 (Kilian et al. 1995). Stress may play an interesting role in this relationship between bacteria and IgA levels. While a number of studies has shown increase in IgA levels following acute stressors (e.g., Bosch et al. 2002, 2003; Brandtzaeg 2007; Trueba et al. 2012) other studies have shown that chronic stressors can lead to a decrease in IgA titers (Henningsen et al. 1992; Drummond and Hewson-Bower 1997). This adds to the complexity involved in the relationship of bacterial contact and asthma (Zhu et al. 2010).
Many of the studies examining the relationship between bacterial infection and asthma have probably recruited a mixture of asthmatic subtypes (Zhu et al. 2010). The hygiene hypothesis postulates specific changes in Th1/Th2 balance dependent on contact with pathogens and infections in early life that lead to protection against atopic conditions. With only about half of the asthma cases being atopic (Holgate 2007) it may be that studies suggesting an increase in the likelihood of asthma development as a result of bacterial infection have involved samples of asthmatics with a substantial number of non-atopic cases.
Regardless of whether the lack of gut bacterial colonization or repeated respiratory infection is related to asthma development, as mentioned above, it appears that there is a relationship between certain bacterial species and asthma (Hilty et al. 2010; Huang et al. 2011). One alternative interpretation of some of these findings is that asthmatic individuals exhibit abnormalities in their mucosa, which changes the microenvironment and bacterial colonization that occurs in their airways. It may also be the case that at an early stage of development the airway epithelium of asthmatics differs from that of healthy individuals and allows for the colonization of different bacterial species. More longitudinal studies of mucosal characteristics and bacterial colonization are needed to elucidate the direction of their relationship.
13.7 The Role of the Microbiome in the Development of Stress Responses and Potential Affective Disorders
New imaging technologies have revealed massive and direct connections between the gastrointestinal tract and the brain (Bohórquez et al. 2014; Tillisch et al. 2008). Recent research also suggests that the intestinal microbiome is involved in the development of the brain (for reviews see: Al-Asmakh et al. 2012; Douglas-Escobar et al. 2013; Collins et al. 2012; Sudo 2012), including the systems that modulate adaptive responses to stress, the HPA axis (Sudo et al. 2004) and the autonomic nervous system (Goehler et al. 2005). This could suggest that the microbiome is implicated not only in the development of asthma, but also in the pathogenesis of affective disorders. Abnormal bacterial colonization is yet another novel mechanism that could explain the comorbidity of asthma and psychopathology (see Fig. 13.3).
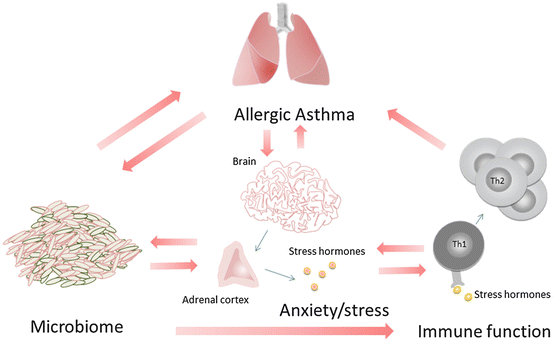
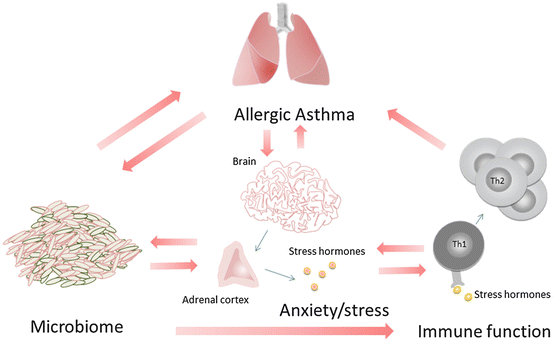
Fig. 13.3
The relationship between affective disorders and asthma is complex and bidirectional. Stress can cause changes in immune function that can result in asthma exacerbation. In turn, the increase in certain cytokines such as IL-1 can cause an increase in cortisol (stress hormones). The microbiome can alter anxiety behavior and in turn the presence of catecholamines (stress hormones) can change bacterial proliferation. The microbiome has also been associated with the development of allergic asthma as it can modulate immune processes relevant in allergic asthma. Hypothetically, stress can also induce changes in the airway bacterial colonization and thereby facilitate inflammatory processes linked to allergic asthma, which in turn may further alter airway microbiome colonization
13.7.1 HPA Axis and the Microbiome
Recent research suggests that bacteria can determine the set-point of the HPA axis (Sudo 2012). The authors compared germ free mice with another group that had gut microbiota, but were pathogen free. They found that restraint stress (immobilization for 1 h) in mice caused an exaggerated ACTH and corticosterone elevation in the germ free mice compared to those with microbiome and pathogen free (corticosterone is the equivalent of cortisol in animals, the main glucocorticoid released in response to stress by the adrenal glands in animals). This was reversed when these mice where given bacteria from the feces of the pathogen free mice and even more so when they were given the bacterial species B. infantis (Sudo et al. 2004). Interestingly, this reversal in the HPA axis responsiveness was only achieved if the bacteria were administered early in the mice development, specifically, only before the age of 6 weeks. The beneficial effects were not observed in mice that were past 14 weeks old. This highlights a possible developmental window that may be particularly relevant for bacteria colonization. The study also indicates that certain bacterial species have a stronger effect on the HPA axis than others. In partial support of these findings, Neufeld et al. (2011) also observed elevations in corticosterone in the serum of mice there were germ-free.
Bacteria can also modulate stress responses by causing alterations in the autonomic nervous system activity. One study found that oral delivery of bacteria Campylobacter jejuni (Gaykema et al. 2004) or Citrobacter rodentium resulted in stimulation of vagal sensory nerves and the central autonomic network (Goehler et al. 2007, see Fig. 13.1b). The mechanism through which bacteria are thought to activate the vagal neurons is through the increased expression of c-Fos protein. C-Fos is a transcription factor considered a marker of neuronal activation as its expression is associated with neuronal firing (increased number of action potentials). There is evidence to suggest that bacterial LPS induces the expression of c-Fos protein in neurons located in the circumventricular organs (structures that lack a blood–brain barrier), the brainstem and pons (Goehler et al. 2005). Similarly oral administration of live bacteria causes an increase in the c-Fos expression in vagal sensory neurons (Goehler et al. 2005). C-Fos expression also increased in the paraventricular nucleus (PVN) of the hypothalamus 6 h after inoculation of B. infantis and this elevation occurred before increases in cytokines were observed (Sudo et al. 2004). In another study rats infected with Salmonella enterica, serovar Typhimurium increased c-Fos protein expression in the PVN and superaoptic nucleus. When these mice were subjected to a subdiagphragmatic vagotomy it reduced the c-Fos expression induced by Salmonella enterica serovar Typhimurium (Wang et al. 2002). These findings suggest that certain bacteria can activate the vagal sensory nerves and therefore may modulate autonomic nervous system activity.
13.7.2 Microbiome and Anxiety Behaviors
The in vitro studies presented in the previous section may imply that bacteria can also be impacted by human stress responses (Belay et al. 2003; Green et al. 2003; Freestone et al. 2007a, b; Diard et al. 2009). Interestingly, recent observations suggest that this relationship is bidirectional, as there is evidence suggesting that bacteria influence autonomic nervous system and HPA axis reactivity which can result in changes in anxiety levels and eating behavior (Alcock et al. 2014, see Fig. 13.3). There is evidence that bacteria can induce cravings for certain food (Alcock et al. 2014). In the fruit fly (Drosophila melanogaster) the bacteria Lactobacillus plantarum in the gut can determine mate selection (Sharon et al. 2010). There is other evidence from animal studies that gut microbiota can also modulate inflammatory pain responses. Amaral et al. (2008) found that germ-free mice had reduced pain measured by recording the hindpaw flexion reflex in response to inflammatory pain. Studies in mice also suggest that bacteria modulated psychological characteristics of the host, such as anxiety behavior (Neufeld et al. 2011; Heijtz et al. 2011). One study found that mice free of bacteria exhibited no anxiety behaviors compared to those that had some bacteria but no pathogenic species (Heijtz et al. 2011). The presence of certain bacteria altered hormone levels such as adreno-corticotrophic hormone (ACTH; Sudo et al. 2004). Germ-free mice have also been found to have elevated noradrenaline, dopamine and serotonin in their striatum when compared to mice that had no pathogenic germs (Heijtz et al. 2011). Another study compared germ free mice and mice that were free of pathogenic bacteria (Neufeld et al. 2011) and found that germ free mice exhibited less anxiety behavior than mice that had a pathogen-free microbiome. The altered behavior of the germ free mice was also accompanied by an increase in brain-derived neurotrophic factor (BDNF) and a decrease in serotonin 1 A receptor expression in the hippocampus. These results suggest that gut microbiome causes changes in anxiety behavior which are linked to alterations in neuronal processes at a molecular level. This also elucidates some of the molecular mechanisms underlying the relationship between gut bacteria and host behavior. Some research has found that the striatum of germ-free mice, compared to normal mice, has higher levels of synaptophysin and PSD95, which are proteins that have an important role in synaptogenesis (Heijtz et al. 2011). Yet another possibility is that anxiety behaviors might be augmented through increases in certain cytokines that are produced as a result of abnormal bacterial colonization. Specifically, there is evidence that bacterial endotoxin and/or IL-1 can increase serotonin in the hippocampus and the medial prefrontal cortex in mice (Rook and Lowry 2008).
One important distinction worth noting is that germ-free mice are distinct from mice that have had dysbiosis, in which the composition of bacteria changes as a result of antibiotic treatment or infection. Studies suggest that mice with dysbiosis (caused by infections by pathogenic bacteria) that had to be treated with antibiotics showed an increase rather than decrease in anxiety behaviors (Lyte et al. 2006; Bercik et al. 2011). A study conducted by Lyte et al. (2006) suggests that anxiety behaviors caused by pathogenic bacteria Citrobacter rodentium might be mediated by vagal sensory nerve stimulation. Another study found that innoculating mice that had an altered gut epithelial barrier with human Bacteroides fragilis (a commensal bacteria) reduced abnormal sensorimotor behaviors and anxiety (Hsiao et al. 2013). Research overall suggests that bacteria interact with both the hormonal stress and the immune system, therefore changing the dynamics between stress and immune processes, with potential consequence for host behavior and inflammatory conditions such as asthma.
13.7.3 Microbiome and Depression
There is evidence that the microbiome can possibly modulate depressive symptoms (Wang and Kasper 2014). The presence of certain bacteria (such as Proteobacteria) can be related to dysphoria, which may have the function of motivating the host to eat certain foods that favor their proliferation (Alcock et al. 2014). Memory impairment, which is observed in individuals with major depressive disorder (Snyder 2013), could also be linked to changes in commensal bacteria (Gareau et al. 2010). There have been several studies showing that infections in general can induce symptoms of depression including lethargy, loss of energy, difficulty concentrating, and decreased appetite among others, as a result of the sudden increase in inflammatory cytokines (Maes 1995; Dantzer et al. 2008). Bacteria could also modulate depression through disruptions in the HPA axis responses as detailed above (Forsythe et al. 2010).
Depression is characterized by disruptions to the serotonergic system (Ogilvie et al. 1996) and alterations in BDNF expression (Martinowich et al. 2007). Both serotonin (Forsythe et al. 2010) and BDNF expression can be modulated by certain bacteria (Sudo et al. 2004; Bercik et al. 2011). One study found that germ free mice that were administered probiotics had a threefold increase in serotonin (Forsythe et al. 2010). There is also evidence that the germ free mice have a decrease in serotonin 1 A receptor expression in the hippocampus (Neufeld et al. 2011). Furthermore germ free mice showed a reduction in BDNF in the hippocampus and cortex (Sudo et al. 2004). In another study, the administration of antimicrobials to specific pathogen free mice changed gastrointestinal microbiota, increased BDNF expression in the hippocampus, and increased exploratory behavior (Bercik et al. 2011). There is also evidence that norepinephrine and serotonin levels in the cortex and hippocampus are reduced in mice that are germ-free compared to mice with microbiome but free of a specific pathogen (Forsythe et al. 2010). Intestinal bacteria produce GABA, which is known to interact with the serotonergic and BDNF systems and is linked to depression and anxiety symptomatology (Kalueff and Nutt 2007). Lactobacilli in particular are among the bacteria that produce GABA (Forsythe et al. 2010) and when ingested it can modulate GABA receptor expression through the vagus nerve (reducing them in the prefrontal cortex and amygdala, and increase in the hippocampus; Bravo et al. 2011).
Like depression, asthma is also associated with changes and disruption of several neurotransmitter pathways, specifically serotonin (Lechin et al. 2002) and BDNF (Nockher and Renz 2006). However, it seems that serotonin levels in asthmatics are high, which is in direct contrast to depressed individuals who have low serotonin levels. These abnormal levels might be related to abnormal activation of the NFkB pathway (in both asthma and depression). In depression there is an increased activation in NFkB and in atopic conditions NFkB might be inhibited by glucocorticoid use. However, interestingly there is evidence that a selective serotonin reuptake inhibitor may reduce asthma symptoms (Van Lieshout et al. 2009). BDNF levels are altered in both asthma and depression, however, asthmatics express high levels of BDNF (Nockher and Renz 2006) in contrast individuals with depression who express low levels (Lechin et al. 2002). One reason for these discrepancies could be that BDNF levels in patients with depression are usually determined centrally whereas in asthmatics BDNF is measured in the airways. There are many other pathways that could serve to explain the relationship between asthma and depression, such as aberrant levels of different cytokines (IL-1ß and TNF-α), prostaglandins, neuropeptides (such as tachykinins) among many others (Van Lieshout et al. 2009). The observed instances of microbiome involvement in the modulation of key neurotransmitters suggest that host-microbiome interactions are important modulators of neutrotransmitter and neuromodulator expression relevant to asthma and depression, although their contribution to development and/or exacerbation of theses conditions is not yet ascertained. Further research on the role of bacterial colonization in the comorbidity of depression and asthma is therefore warranted.
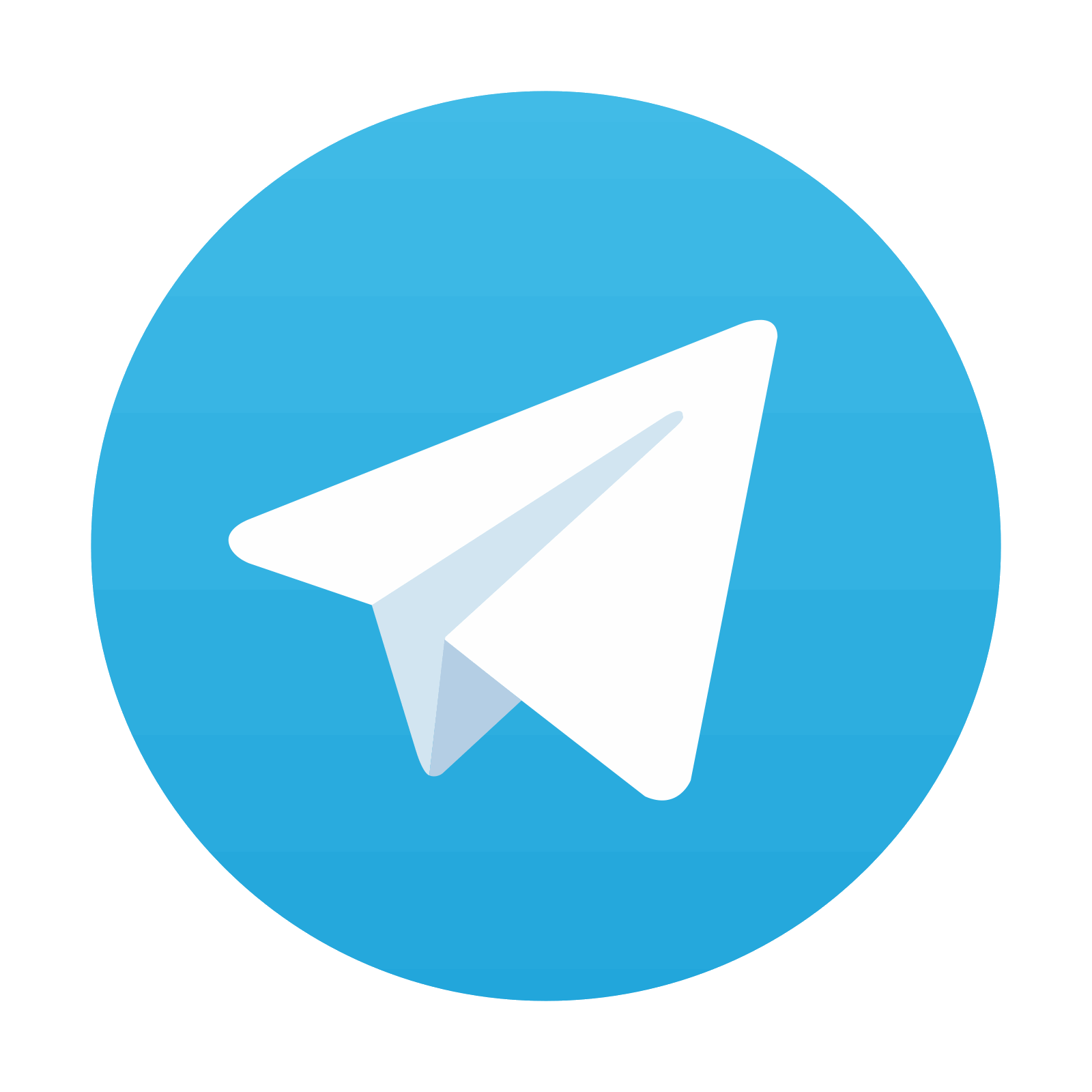
Stay updated, free articles. Join our Telegram channel
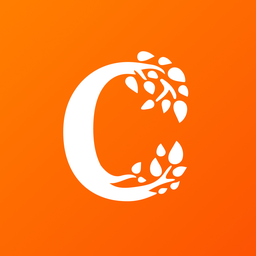
Full access? Get Clinical Tree
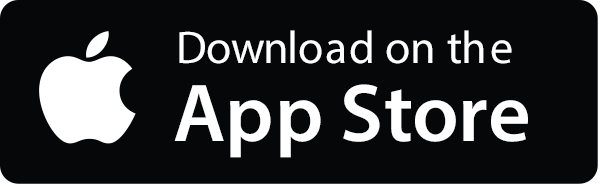
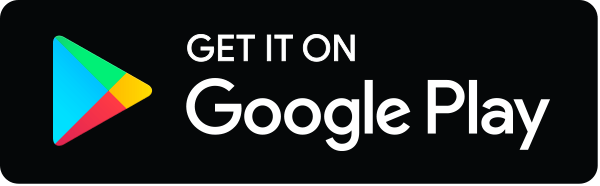