Fig. 15.1
The microbiome industrial age. Host genetics, life style and available nutrients (diet) are the main determinants of the composition and diversity of the intestinal microbiome. This lays the basis for production of microbial metabolites that act as effector molecules, yielding substrates for downstream host metabolic reactions and regulating host physiology. This, at least in part, is orchestrated by epigenetic mechanisms. In addition, the host immune system is stimulated by intestinal microbes and immune signals are relayed to other organs, including the brain. Together with direct nervous connections, these mechanisms influence brain functions, including anxiety, neurodevelopment, and pain perception. Therefore, the microbiome is an important part of a feedback loop, mediating of gene-environment interactions. Abbreviations: EEC enteroendocrine cell, DC dendritic cell, ENS enteric nervous system
Nonetheless, only a handful of bioactive microbiota-dependent metabolites have been identified to date, with very few known to be involved in maintaining the host-microbial homeostasis (Nicholson et al. 2012). Unravelling the complex microbiota-metabolic processes and products is coming to grips with the difficulty in culturing the majority of the intestinal microbiota under standard laboratory conditions. The fact that metabolites produced by one bacterium can be utilized or modified by others adds to the complexity of the identification of microbiota-produced metabolites. Moreover, the classification of the identified metabolites as microbiota- or host-derived is another challenge since the majority of metabolites are shared between pro and eukaryotes (Peregrin-Alvarez et al. 2009). Linking the intestinal microbiota composition to functionality requires bottom-up as well as top-down approaches with combined application of in vitro, humanized mouse models and human intervention trials (Van Duynhoven et al. 2011). In a recent study, a metabolomics strategy was developed to facilitate the characterization of microbiota-dependent metabolites and describe which molecules are formed from which bacterial biotransformation reactions (Sridharan et al. 2014). Among the identified metabolic reactions, amino acid metabolism represented the largest group that require the microbiota for their synthesis and metabolism, as illustrated by their low abundance or absence in the caecum of GF mice. The findings of this study are compatible with previous reports plasma and urine metabolites in GF and antibiotic-treated rodents (Wikoff et al. 2009; Zheng et al. 2011).
15.4.1 Microbiota-Dependent Metabolites
TRIMETHYLAMINE (TMA) is the product of microbiota-dependent choline (essential dietary nutrient) metabolism (Dumas et al. 2006). TMA is identified only in the urine samples of ex-GF mice indicating it is exclusively produced by the gut microbiome (El Aidy et al. 2013a; Claus et al. 2008). A potential link between the intestinal microbiota, dietary choline, and cardiovascular disease risk has been suggested, where increased metabolites of the dietary lipid phosphatidylcholine have been observed in the blood of patients suffering from myocardial infarction or stroke compared to that of normal individuals (Wang et al. 2011). TMO is also associated with bad breath as seen in patients with the genetic disease trimethylaminuria that is associated with a mutation of the liver enzyme flavin-containing monooxygenase 3 (FMO), which oxides TMO into trimethylamine-N-oxide (TMAO) (Mitchell and Smith 2001). Recently, TMO was shown to be involved in species-specific social communication, via its olfactory receptor, trace amine-associated receptor 5 (TAAR5) (Li et al. 2013). Unlike in humans, TMO is detected in mouse urine in much higher levels, where it serves as a strong attractive odour source to ensure that mating and other social behaviours are properly directed in a concentration-dependent manner. Indeed, depletion of FMO or depletion of the TAAR5 receptor was linked with decreased odour attraction in mice suggesting an impact of TMO on behaviour and brain function.
BILE ACIDS integrated metabolism in mammals represent an intriguing example of the inter-kingdom signalling between the host and microbiota. Bile acids function by facilitating the metabolism of dietary fat and the absorption of fat-soluble vitamins and cholesterol. Biotransformation of about 5–10 % of bile acids occurs through degradation by the major groups of intestinal bacteria, including Bacteroides, Eubacterium, Lactobacillus, Escherichia and Clostridium via bile salt hydrolase enzymes (Ridlon et al. 2006). Reports from GF and antibiotic treated rodents described the crucial role of the intestinal microbiota in the metabolism of bile acids. GF mice have higher levels of phospholipids in their liver and higher levels of bile acids in gut tissue, indicating that the gut microbiota is a key regulator of bile acid metabolism (Swann et al. 2011). Altered expression in genes associated with cholesterol, steroid, and bile acid synthesis as well as altered conjugated bile acid was characteristic in multiple body compartments of these animals.
Bacterial degradation of bile acids involves the deconjugation of taurine- and glycine-conjugated bile acids to unconjugated free bile acids, which in turn undergo reabsorption, mainly by bile acid transporters in the ileal epithelium but also by passive absorption throughout the intestine (Dawson et al. 2009). Through their action on bile acid metabolism, the intestinal bacteria can indirectly affect several pathways involved in energy and lipid metabolism, bile acid synthesis and transport, lipid and carbohydrate metabolism, and even the regulation of intestinal innate immunity. This action occurs via the ligation of bile salts with one of the nuclear hormone receptors; the farnesoid X receptor (Vavassori et al. 2009).
Impaired bile acid metabolism has been linked with microbial dysbiosis (Duboc et al. 2013), which in turn alters the capacity of the gut community to modify bile acids (Ogilvie and Jones 2012) resulting in impairment of the enterohepatic flow, which is required for regulation of bacterial populations and growth rates to achieve the normal balance of bacteria throughout the GI tract (Ogilvie and Jones 2012). Impaired enterohepatic flow leads also to digestion and absorption of fat-soluble nutrients and is associated with impairment in bile-acid amino transferase (BAAT), which catalyses the final reaction in the formation of the primary conjugated bile acids. Intriguingly, in a case-study of an old female who had a genetic deficiency in BAAT, showed unique pattern of elevated levels of taurine and glycine bile acids, CNS dysfunction and intestinal dysbiosis with elevated levels of Fusobacteria. Correction of microbial population and improved CNS functions were rapidly produced upon application of BAAT replacement therapy (Lord et al. 2014).
Bile acids-related microbial dysbiosis has also been linked with hepatic encephalopathy (Bajaj et al. 2013). Hepatic encephalopathy represents an interface for the microbiota signalling to the gut-liver-brain axis. The successful treatment of hepatic encephalopathy with antibiotics suggests the involvement of microbial dysbiosis in the aetiology of the disease. Indeed, microbiota changes in hepatic encephalopathy have been associated with impaired cognition, endotoxemia, and inflammation, where reduced abundance of the taxa Lachnospiraceae, Ruminococcaceae, and Clostridiales XIV and increased Enterobacteriaceae and Streptococcaceae were reported (Bajaj et al. 2012). This dysbiosis is related to a reduction in hepatic bile acid synthesis, which in turn leads to more release into the intestine, and subsequent systemic inflammation. Dysbiosis in hepatic encephalopathy results also in an increase in gut-derived products such as ammonia, endotoxin, inflammatory cytokines, and bacterial DNA into the systemic circulation via the mal functioning liver (Zapater et al. 2008). Subsequently brain dysfunction including cognitive impairment and neuro-inflammation has been associated with HE (Bajaj 2014).
In summary, bile acids represent an intriguing example of co-metabolism between the gut microbiota and host and suggest that modulation of bile acid metabolism activity in the microbiota may be an effective target in the treatment of obesity and metabolic syndrome (Joyce et al. 2014).
PHENOL and phenolic derivatives are produced by the intestinal microbiota, in particular, Clostridium, Bifidobacterium, Bacteroides fragilis and Escherichia coli, from tyrosine (Bone et al. 1976). Around 50–100 mg of volatile phenols are excreted in humans per day, mainly in the form of glucuronide and sulphate conjugates of phenol or 4-cresol (Nicholson et al. 2012). Altered levels of volatile phenols in human urine have been linked to a large array of physiological and pathological conditions, including weight loss and inflammatory bowel disease (IBD). 4-Cresol produced by Clostridia was detected at significantly higher concentrations in the urine samples of children with autism spectrum disorders (ASD) and in schizophrenia and treatment with antibiotics against Clostridia species improved the autistic symptoms (Zheng et al. 2011). The underlying mechanism by which phenolic compounds produced by Clostridia contribute to the markedly altered behaviour in autism and other neuropsychiatric diseases is proposed to involve the inhibition of the conversion of dopamine to norepinephrine (Shaw 2010). Elevated levels of dopamine not only cause abnormal behaviour but also in severe brain damage.
Notably, 4-cresol (4-methylphenylsulfate) shares structural similarity with another microbial-dependent metabolite: 4-ethylphenylsulfate (4EPS) (Hsiao et al. 2013). Like, 4-cresol, 4EPS was found to induce ASD related behavioural abnormalities when injected in naïve mice. Moreover, 4EPS was dramatically elevated in serum levels of offspring of maternal immune activation (MIA), a mouse model which exhibits features of ASD. 4EPS is proposed to be produced by Lachnospiraceae family of Clostridia and ingestion of Bacteroides fragilis was elegantly shown to restore the serum levels of 4EPS to normal.
INDOLE is exclusively produced by the intestinal microbiota, which converts tryptophan into indole, pyruvate and ammonia by the bacterial tryptophanase enzyme (Lee and Lee 2010). Indole regulates gut immune cells and is proposed as a potential treatment of IBD via its immunomodulatory and anti-inflammatory effects on intestinal epithelial cells, which are central regulators of gut homeostasis (Bansal et al. 2010). Indole can be further modified into indole-2-acetic acid (IAA) and the neuroprotective molecule; indole-3-propionic acid (IPA). Incubation of human large intestinal content with tryptophan and indolelactate resulted in the production of IPA in vitro (Smith and Macfarlane 1997). In vivo, IPA was detected in the plasma and cerebrospinal fluid (Young et al. 1980). Intriguingly, IPA was shown to completely protect primary neurons and neuroblastoma cells against oxidative damage and death caused by exposure to Alzheimer β amyloid protein, via inhibition of superoxide dismutase, or by treatment with hydrogen peroxide (Chyan et al. 1999). Collectively, the gut microbiota appears to sequester tryptophan from the diet and alter its metabolites in the host, resulting eventually in altered brain levels of neuropeptide that affect the brain function. In fact, the key microbial enzyme tryptophan decarboxylase, which converts dietary tryptophan to the neuropeptide tryptamine, has been recently identified (Williams et al. 2014). The enzyme was found to be present in several bacteria that colonize about 10 % of the human population. Altered levels of tryptamine in urine have been used in diagnosis, where low levels of tryptamine in urine were detected in patients with severe depression (Coppen et al. 1965). Tryptamine also stimulates the release of serotonin from enterochromaffin epithelial cells (Takaki et al. 1985) and is a key regulator of the gut motility and secretion (Turvill et al. 2000).
VITAMINS B12 and K are synthesized by the intestinal microbiota that are also capable of producing most of the water-soluble B vitamins, such as biotin, cobalamin, folates, nicotinic acid, panthotenic acid, pyridoxine, riboflavin and thiamine in humans (Hill 1997). Vitamin B12 (cobalamine), which is essential for the development of the nervous system (Dror and Allen 2008), is produced by L. reuteri (Santos et al. 2008). Vitamin K, which has a modulatory role in cognition (Ferland 2012), is produced by several bifidobacterial strains (Leblanc et al. 2013). Microbially produced vitamins are taken up in the colon unlike the dietary vitamins, which are adsorbed in the proximal tract of the small intestine (Said and Mohammed 2006).
SCFAs are produced by the fermentation of complex polysaccharides in the colon, which require the cooperative action of different microbial population groups, including various species of the anaerobic Fermicutes; Clostridial clusters IV and XIVa such as Eubacterium, Roseburia, Faecalibacterium, and Coprococcus species (Flint et al. 2012; El Aidy et al. 2013c). Complex carbohydrates are broken down to mono- and oligomeric compounds that can be broken down further to the SCFA acetate, propionate, and butyrate as well as to carbon dioxide (CO2,) and molecular hydrogen (H2). Other important intermediates are lactic acid, ethanol, succinic acid, and formate that are also degraded to SCFA, CO2, and H2 (Blaut and Clavel 2007). SCFAs are the preferred source of energy for colonocytes and are also considered as a source of energy to the brain, where they can cross the blood-brain barrier to be taken up by the glial cells and, to lesser extent, the neurons (Karuri et al. 1993). Subsequently, continual secretion of SCFAs by the intestinal microbiota may result in long-lasting effects on gene expression patterns that are necessary for appropriate neuronal development and function even though the effects of SCFAs that cross the blood-brain barrier under physiological conditions may be marginal.
In adequate levels, propionate was shown to improve insulin sensitivity and lower serum cholesterol levels (Hosseini et al. 2011). Nonetheless, studies conducted in rodents suggested that excessive levels of propionate are detrimental to the host health and behaviour, indicating it is crucial to maintain a balanced microbial community. Indeed, higher levels of Bacteriodetes and Clostrial species were detected in patients with ASD (Hosseini et al. 2011). Intraventricular injection of propionate in rats was associated with impaired social behaviour and altered brain phospholipid composition in a way similar to symptoms observed in ASD patients (Thomas et al. 2012a). The link of propionate with ASD is due to its ability to alter the levels of the neuropeptides; serotonin, glutamate and dopamine (El-Ansary et al. 2012). Butyrate has been the focus of many in vitro and in vivo studies, which unravelled its impact on the human physiology. Butyrate regulates energy homeostasis, stimulate leptin production in adipocytes (Musso et al. 2011). Moreover, levels of the neuropeptide glucagon-like peptide-1 (GLP-1) were induced in response to butyrate, resulting in modulation of insulin secretion, lipid and glucose metabolism, and food intake (Burcelin et al. 2007). Butyrate was also reported to have profound effects on mood and behaviour, where it elicited antidepressant effects in murine brain (Schroeder et al. 2007). Through their effect on gastric motility and intestinal transit stimulation, SCFAs resulted in an elevation in serotonin release as reported in an in vitro colonic mucosal system (Grider and Piland 2007). SCFAs have been reported to alter the expression of brain derived neurotrophic factor (BDNF), stimulate the sympathetic nervous system, and may influence social behaviour in rodents (Macfabe et al. 2011; Schroeder et al. 2007). Acetate, is a major substrate for acetyl CoA synthesis and, through the histone acetyltransferase (HAT) activity, is involved in the process of acetylation of histone tail lysine residues (Stilling and Fischer 2011). Thus, SCFAs enhance histone acetylation by inhibition of HDACs on the one hand and increased availability of HAT substrate on the other hand. In fact, several in vitro and in vivo models for learning and memory and neurodegenerative diseases illustrated that enhanced histone acetylation facilitated long-term memory consolidation and neuroprotection/-regeneration in a numerous in vitro studies and animal (Peleg et al. 2010; Govindarajan et al. 2011).
In summary, the microbial derived SCFAs are essential contributors to host metabolism through their action as an energy source or through balancing host gene expression throughout brain development and, more dynamically, in adulthood (Macfabe 2012; Gundersen and Blendy 2009; Thomas et al. 2012b; Selkrig et al. 2014).
In addition to SCFAs, the intestinal microbiota is capable of affecting the availability of dietary sources of methyl-group donors by modulation of one carbon metabolism and thereby potentially affecting host DNA and histone methylation (Cabreiro et al. 2013). Spermidine, a ubiquitous polyamine, has also been also shown to be produced by the intestinal microbiota (Noack et al. 2000). Spermidine has beneficial effects on ageing and age-associated memory impairment (Gupta et al. 2013), which may in part be mediated by an alteration in histone acetylation (Das and Kanungo 1979).
15.4.1.1 Neuroactive Chemicals
It is well recognized that a variety of the intestinal microbiome, in particular, lactic acid bacteria, has the capacity to produce several molecules with neuroactive function including gamma amino butyric acid (GABA), acetylcholine, catecholamines, and serotonin. For example, GABA is produced by species of Lactobacillus and Bifidobacterium, with L. brevis and B. dentium being the most effective producers (Barrett et al. 2012). GABA appears to protect its producing bacteria from the gastric acidity (Higuchi et al. 1997). Intriguingly, in vivo experiments illustrated a modulatory effect of the microbiota-derived GABA on the host neural cells (Bravo et al. 2011). Ingestion of L. rhamnosus JB-1 resulted in differential expression of the GABA A and B receptor subunits, which are responsible for maintaining normal fear and mood responses.
Several species of Escherichia, Bacillus, Lactococcus, Lactobacillus, and Streptococcus are capable of producing the catecholamines; dopamine and norepinephrine (Shishov et al. 2009) in quantities that are thought to be higher than the catecholamines content in the human blood (Wall et al. 2014). The intestinal lumen of GF mice has lower levels of biologically inactive dopamine and norepinephrine than their conventionally raised counterparts. The substantial elevation of the luminal free catecholamines was associated with the colonization of gut microbiota, which has abundant beta-glucuronidase activity (Asano et al. 2012). Moreover, dopamine receptor expression is significantly altered in the gut and circulation but also in the brain during development (El Aidy et al. 2013b; Diaz Heijtz et al. 2011; Neufeld et al. 2011). Catecholamines represent the major class of neurotransmitters, which are involved in various neurological functions including emotion and endocrine regulation as well as cognition and memory processing (Kobayashi 2001). Subsequently, disturbance in catecholamines levels have been linked to several neurological disorders such as Parkinson disease (Calabresi et al. 2013), Alzheimer’s disease (Robertson 2013) and major depressive disorders (Hamon and Blier 2013).
Acetylcholine is produced by different strains of Bacillus and Lactobacillus, in particular L. plantarum (Girvin and Stevenson 1954). Acetylcholine is crucial in maintaining cognitive function, memory and learning as well as the neuro-inflammatory influx circuit (Olofsson et al. 2012).
Various Streptococcus, Escherichia, Enterococcus, Lactococcus, and Lactobacillus strains produce the neuroactive compound serotonin. Reports from animal experiments showed that ingestion of B. infantis for 2 weeks resulted in elevated plasma levels of the precursor of serotonin; tryptophan (Desbonnet et al. 2008). Moreover, the plasma levels of serotonin were three times higher in conventionally raised mice when compared to GF mice (Wikoff et al. 2009). In contrast, the levels of tryptophan were lower in conventionally raised mice (El Aidy et al. 2014a; Clarke et al. 2013). It remains to be unfolded how the intestinal microbiome impacts the tryptophan metabolism, presumably through the modulation of the expression level of the catalytic enzyme indoleamine 2,3 dioxygenase (Ido) in the Kynurenine arm, which occurs during immune activation (Moffett and Namboodiri 2003) and is observed in many disorders of both the brain and GI tract (Forsythe et al. 2010) but also transiently during primary gut colonization (El Aidy et al. 2012a). Recently it has been shown that gut microbiota, acting through SCFAs, is an important determinant of enteric serotonin production and homeostasis (Reigstad et al. 2014). The gut microbiota from ex-GF colonized with human gut microbiota and conventionally raised mice significantly increased the colonic rate limiting for mucosal serotonin synthesis; tryptophan hydroxylase (Tph) 1 mRNAs as well as the neuroendocrine secretion gene; chromogranin A, through the action of SCFAs.
Cyclic dipeptides (CDPs), a group of hormone-like compounds, represent another example of inter-kingdom signalling. Bacteria not only use CDPs in communicating with each other, but also in signalling to its host that uses the same molecules to regulate inflammation and induce protective effect in neuronal cells (Bellezza et al. 2014), suggesting that CPDs could have therapeutic value in a range of inflammatory and neuronal disorders.
It remains to be elucidated though why certain members of the intestinal microbiome are capable of producing neurochemicals. Bacteria use these molecules to communicate with each other in a process known as Quorum-sensing (Lyte 2011). It is thus possible that bacteria utilize these chemical signalling molecules in order to communicate with their hosts (Boontham et al. 2008). In fact, these intraluminal neuropeptides are thought to play a crucial role in modulating the ENS through their action on epithelial cells, to eventually influence the central nervous system (CNS), mood and behaviour (Lyte 2011; Dinan et al. 2013). It is thus tempting to view these bacteria as delivery vehicles for neuropeptides, which may be pivotal in the prevention and treatment of certain neurological and psychological disorders. This idea has recently been coined the term “psychobiotics” (Dinan et al. 2013).
15.4.2 Microbial Mimicry of the Host Epigenetic Machinery
Next to modulation of host epigenetics through metabolic activity, several pathogenic bacteria are able to secret effector proteins that mimic eukaryotic epigenetic enzymes and regulators to orchestrate infected cells in their own favour (Bierne and Cossart 2012). So far, these molecular tools have been discovered exclusively in intracellular parasites that interact with host signalling within the intracellular environment. It will be intriguing to search for such capabilities neuron-targeting pathogens as well as non-pathogenic microbes secreting epigenetic modulators that may be transported across epithelia and plasma membranes to affect host cells in a more paracrine manner. Taken together, the above-mentioned examples for inter-kingdom molecular manipulation shows the versatile ways open to microbes to interact with the host’s transcriptional machinery.
15.5 The Gut-Brain Axis: The Pathways for Gut Microbiota to Modulate Brain Function
Although great progress has been made over the last decades in describing the bidirectional interactions between the GI tract, ENS and CNS, novel interest in this field of research has been stimulated by a growing body of intriguing preclinical studies signifying a prominent role of the gut microbiome in the gut-brain dialogue. The modulatory effect of the gut microbiota on the gut brain axis was demonstrated in a number of studies using GF animal models, or modulation of microbiota with antibiotics, faecal microbial transplantation (Bercik et al. 2011a) or probiotics (Bravo et al. 2011). On the molecular level, GF mice show an altered expression of genes involved in neuropeptide production, N-methyl-d-aspartate (NMDA) receptor subunits (Neufeld et al. 2011) and genes involved in brain development and behaviour including altered expression of BDNF in the hippocampus (Diaz Heijtz et al. 2011; Gareau et al. 2011). Interestingly, some of the reported molecular changes in neuroreceptor expression have been associated with altered mood and behaviour. In the absence of a normal gut microbiome, significant changes in adult depression like behaviour (Schroeder et al. 2007), nociceptive responses (Amaral et al. 2008; Rousseaux et al. 2007), stress responsiveness (Gareau et al. 2007) and social development (Desbonnet et al. 2014) have been shown, and these alterations were partially reversed by colonization of the GI tract. The gut microbiota was associated with elevation in anxiety-like behaviour in conventional mice upon ingestion of probiotics such as L. rhamnosus (Bravo et al. 2011), B. longum (Bercik et al. 2011a) and B. infantis (Desbonnet et al. 2010). GF mice have recently been shown to have elevated repetitive behaviours and core social abnormalities in a similar way to that observed in ASD (Desbonnet et al. 2014). These findings are further supported by the correlative studies performed in humans, albeit in relatively small cohorts, which suggested that ASD may be associated with alterations in microbiota composition and metabolism (Critchfield et al. 2011; De Theije et al. 2011; Gondalia et al. 2012; Louis 2012; Macfabe 2012; Ming et al. 2012; Mulle et al. 2013; Douglas-Escobar et al. 2013). As such, the mechanisms underlying the development of ASD are still to be determined. While the cause of these conditions seems to be mostly genetic (including de novo mutations), it is unclear how the genetic information is translated to the behavioural and gastro-intestinal phenotypes associated with ASDs. Interestingly, both epigenetic mechanisms, including ncRNAs, (Grafodatskaya et al. 2010; Hall and Kelley 2014; Helsmoortel et al. 2014; Mellios and Sur 2012; Miyake et al. 2012; Schanen 2006; Wilkinson and Campbell 2013; van de Vondervoort et al. 2013; Ziats and Rennert 2013) and changes in the microbiota (Cao et al. 2013; Desbonnet et al. 2014; De Theije et al. 2014; Hsiao et al. 2013; Gorrindo et al. 2012; Kang et al. 2013; Peters et al. 2014) have been suggested to be involved in this process (Stilling et al. 2014a).
Additionally there is evidence for a contribution of genetic and environmental risk factors as well as a strong effect of microbial composition in visceral hypersensitivity associated with IBS (Ford et al. 2014; Fukuda and Ohno 2014; Shankar et al. 2013). Probiotic-based therapies have been used to decrease visceral hypersensitivity in preclinical models (McKernan et al. 2010) and in human trials (Clarke et al. 2012). Moreover, in line with the suggestion that epigenetic mechanisms are at the heart of the clinical manifestation of IBS (Dinan et al. 2010) Greenwood Van-Meerveld demonstrated amelioration of the stress-induced increase in visceral pain sensitivity by administering the epigenetic drug TSA (an HDAC inhibitor) directly to the brain (Tran et al. 2013). Future studies should be targeted at clarifying how microbes and their metabolites modify epigenetic pathways relevant to the origin and central processing of visceral pain.
Much of the attention directed towards the gut microbiota in regard to the gut-brain axis focuses on the ability of these commensals to potentially recruit the HPA axis to regulate the stress response and studies performed in GF mice illustrated an increase in the activity of HPA axis as measured by blood corticosterone or adrenocorticotrophic hormone levels (Neufeld et al. 2011; Clarke et al. 2013; Sudo et al. 2004). In contrast, gut neuropeptides have received considerably less attention in this regard although they can both be controlled by and influence the activity of the microbiome. Neuropeptides are produced in the gut in response to the microbial residents (Lyte and Cryan 2014), analogous to the neuroactive chemicals produced by the intestinal microbiota as discussed earlier. Specific members of gut neuropeptides can function at multiple levels of the brain-gut axis to guide not just local events in the GI tract but also distally at the level of the CNS to influence brain and behaviour. Bacteria can actively recognize the host neuroendocrine hormones and several studies showed the rapid growth and enhanced surface attachments (through biofilm formation) of these microorganisms when cultured in media containing small amounts of the catecholamines (Lyte et al. 2003). These findings were the groundwork of the theory of “microbial endocrinology”. Intriguingly, when mice were administered a neurotoxic drug that caused the release of norepinephrine from the catecholaminergic neurons in the gut and other autonomic sites, the number of the gut populated gram negative bacteria are massively increased. These changes reversed as the catecholaminergic nerves regenerate within 2 weeks. The emerging evidence of the dramatic impact that the altered neuroendocrine environment can have on the gut microbes suggest that even minor changes in the levels of neuropeptides can lead to dysbiosis.
Bacterial components and by-products that come in contact with the gut epithelium stimulate a group of gut epithelial cells, enteroendocrine cells (EECs), to produce several neuropeptides such as peptide YY, neuropeptide Y, cholecystokinin, glucagon-like peptide-1,2, and substance P (Furness et al. 2013). Through receptors expressed on EECs, neurons of submucosal and myenteric ganglia as well as on enteric leukocytes, the gut senses the bacterial by-products (Samuel et al. 2008; Nohr et al. 2013). Upon their secretion by EECs, neuropeptides presumably diffuse throughout the lamina propria, which is occupied with a variety of immune cells, until they reach the blood stream or act on the vagal nerve or intrinsic sensory neurons (Cummings and Overduin 2007; Okano-Matsumoto et al. 2011) but the exact mechanisms and whether the neuropeptides have a direct contribution in the bidirectional communication between the microbiota and CNS are still obscure. Recently, Bohorquez et al. suggested an accurate temporal transfer of the sensory signals originating in the gut lumen with a real-time modulatory feedback onto the EECs. They demonstrated a direct communication between EECs and neurons innervating the small intestine and colon that alternates the paracrine transmission (Bohorquez et al. 2015).
The interplay between gut microbiota and the gut-brain axis can also occur through the autonomous (enteric, sympathetic and parasympathetic, which includes the vagus nerve) nervous system (ANS), sensory nerves, immune mediators and alterations in the gut functionality (motility, secretion, and permeability) (Mayer 2000; Holzer and Farzi 2014).
The ANS was shown to influence the size and quality of the mucus layer, and subsequently on the biofilm structures, where the majority of the colonic microbiota reside (Rhee et al. 2009). Throughout the GI tract, the community structure of commensals is likely to be dramatically altered in response to disturbed gut motility and increased intestinal permeability, which is also associated with small intestinal bacterial overgrowth (SIBO) (Van Felius et al. 2003). GI dysfunction, in particular constipation, often precedes the onset of motor symptoms by years in the aetiology of Parkinson’s disease (PD), since both ENS and parasympathetic nerves are amongst the structures earliest and most frequently affected (Derkinderen et al. 2011). A recent study carried out in PD patients illustrated that the intestinal microbiome is altered in PD and is related to motor phenotype (Scheperjans et al. 2014). When the faecal microbiomes of 72 PD patients were compared to those of 72 control subjects, the abundance of Prevotellaceae was found to be dramatically reduced in faeces of PD patients. Moreover, the relative abundance of Enterobacteriaceae was positively associated with the severity of postural instability.
Several of the studies investigating the impact of the intestinal microbiota on behavioural and neurophysiological changes investigated the contribution of the vagus nerve. Indeed, vagotomy eliminated some of the effects found in studies on mice fed with probiotics or pathogens (Bravo et al. 2011; Bercik et al. 2011b). The modulated stimulation of the vagal pathways could occur as a result of altered gut motility or neurochemicals produced by the intestinal microbiota. Nonetheless, the exact modalities of how the vagus interacts with the microbiota to induce such effects are obscure. Other experiments suggested also that at least some of the observed effects of the gut microbiota on behavioural changes are functionally independent of the vagus or other autonomous pathways (Bercik et al. 2011a). Collectively, these findings indicate that the vagus nerve is an important, but not the only mediator in the microbiota-gut-brain axis.
15.6 The Microbiome as a Pivotal Component in the Psychoneuroimmunology Network
The intersection of neurology and immunity has its roots in modern science and the involvement of the nervous system in regulating the whole immune system and vice versa has led to the establishment of the field of psychoneuroimmunology (Ader and Kelley 2007). A growing body of evidence shows a significant contribution of immune signalling in normal brain function as well as during ageing and in the context of neurodegenerative diseases. This bidirectional cross talk is regulated by a network of signalling pathways, which involves (but not exclusively) the HPA axis and the ANS (Soliman et al. 2013; Lampron et al. 2013; Villeda et al. 2011; Collins et al. 2012). However, we are only beginning to fully appreciate the widespread interaction of the intestinal microbiota with the immune system and the neuroendocrine system, which strongly suggest the microbiota to be a decisive component in the psychoneuroimmunology network (El Aidy et al. 2014b). Intestinal microbiota may coordinate the neuroendocrine-immune dialogue via several mediators including epithelial cells, (mucosal) immune cells as well as peripheral neurons (Forsythe and Kunze 2013; El Aidy et al. 2012b). For example, the ANS affects epithelial mechanisms involved in the intestinal immune activation through stimulation of the immune cells residing in the lamina propria to produce antimicrobial peptides against the enteric bacteria or via modulating the access of the microbiota to the immune cells (Alonso et al. 2008). The latter effect has been linked to changes in the gut permeability and increased translocation of commensals under stressful conditions (Keita et al. 2010). In fact, the context of the “leaky gut” hypothesis is another mechanism for inducing immunomodulatory effects in disorders of the brain-gut axis that involves the intestinal microbiota. Chronic stress, for example, has been shown to increase the gut permeability to bacterial peptides (Santos et al. 2001) and the adverse effects were reversed by probiotics (Ait-Belgnaoui et al. 2012; Zareie et al. 2006). These findings were supported by data from human studies, which indirectly suggest increased bacterial translocation in stress-related psychiatric disorders such as depression (Maes et al. 2012).
In healthy conditions, the majority of the gut microbiota is kept at bay, with no direct contact with the host cells, suggesting that the microbial products and neuroactive chemicals are most likely responsible for transferring the microbial signalling to the host. However, in states of disease and microbial dysbiosis, several pathobionts (potentially pathogenic bacteria, which are part of the normal microbiome community in a state of homeostasis) are capable of invading host tissues and can even live in intracellular vacuoles to manipulate host cells directly, thereby activating the mucosal immune cells and associated parts of the ENS (Lievin-Le Moal and Servin 2013). In fact, a similar mechanism could be orchestrating the early life modulation of the immune-neuroendocrine network following microbial colonization. Some animal studies illustrated that specific pathobionts activate mucosal immune process for bacterial sampling by transient breaching the epithelial barrier in order to minimize their exposure to the systemic immune system. This initial process of immune activation is associated with stimulation a variety of immune pro-inflammatory and regulatory immune components as well alter the expression of intestinal neuropeptides (El Aidy et al. 2013b, 2014a; Galindo-Villegas et al. 2012; Mazmanian et al. 2005). Elimination of the penetrant bacteria would require the engagement of immune and neuroendocrine components. Hence, this initial close but regulated contact could benefit the host by strengthening its gut barrier and conferring protection against true invading pathogens. Intriguingly, microbiota-immune-neurological communication is thought to be directed by neuropeptides, where many immune cells travel through the blood and when they come within scenting distance of a given neuropeptide they begin to chemotaxically orient toward it, and then communicate with other immune cells (Straub et al. 2006). Indeed, several neuropeptides direct the migration of immune cells, including immature DCs migration to lymph nodes at the start of a local immune response, as well as during the initial microbial colonization, via α1 adrenergic receptors, emphasizing the early involvement of the SNS (Maestroni 2000). The interaction between specific type of immune cells, ENS and microbiota was recently shown to also regulate the GI motility (Muller et al. 2014). This regulation occurs when a subset of macrophages, named muscularis macrophages (MMs) that reside in close proximity to the myenteric plexus and intestinal cells of cajal (ICC) is stimulated by the intestinal microbiota to produce signalling molecules, which in turn stimulates the ENS (Kunze and Furness 1999; Muller et al. 2014). Whether the regulatory mechanisms employ signals provided by EECs remains to be elucidated.
Stimulation of the immune response by the gut microbiota induces the production of pro-inflammatory cytokines, which can inhibit the release of norepinephrine from noradrenoceptor axon terminals, via the induction of nitric oxide (Ruhl and Collins 1997). Elevated immune responses have also been linked with decreased activity of L-DOPA decarboxylase, the enzyme that converts L-DOPA to norepinephrine, and subsequently reduced levels of norepinephrine as observed in both inflamed and non-inflamed colonic mucosae of Crohn’s patients (Magro et al. 2002). In fact, increased concentrations of inflammatory cytokines are known to circumvent the mechanisms of action of conventional antidepressants, suggesting that inhibition of the inflammatory cytokines would reduce depressive symptoms. This assumption was supported by the results from a placebo-controlled, randomized clinical trial showing that the TNF antagonist infliximab reduces depression symptoms in a subset of patients with high baseline inflammatory biomarkers (Raison et al. 2013).
Moreover, immune stimulation with non-pathogenic bacteria was shown to activate a functionally and anatomically distinct subset of serotonergic neurons, in the interfascicular part of the dorsal raphe nucleus of mice, which are different from the subset of serotonergic neurons activated by anxiety-inducing stimuli or uncontrollable stressors (Lowry et al. 2007). Activation of the peripheral immune system with antigens derived from the nonpathogenic bacterium Mycobacterium vaccae resulted in increased serotonin metabolism within the ventromedial prefrontal cortex, with temporal reductions in immobility in the forced swim test, indicative of alters stress-related emotional behaviour. Likewise, treatment with serotonergic antidepressant drugs prevents the onset of depressive symptoms in patients with irritable bowel syndrome (IBS), and in patients receiving treatment with interferon (Capuron and Miller 2004; Felger et al. 2013). Collectively, these findings suggest that serotonergic systems may be a plausible route by which the gut microbiota coordinates the immune-neuroendocrine communication. Whether the results to date reflect a causative or reactionary response is yet to be elucidated.
The principle vagal neurotransmitter, acetylcholine, is another important mediator in shuttling information between the microbiota and host nervous and immune systems, all of which can produce and respond to neuropeptides. Acetylcholine attenuates the release of a plethora of pro-inflammatory cytokines with no effect on the anti-inflammatory IL-10 (Borovikova et al. 2000), and is released from a subset of CD4 +T cells that transfer the signal to other immune cells through the activation of α7 nicotinic acetylcholine receptors on macrophages (Wang et al. 2003). Acetylcholine is also produced by a specific type of T cells known as ChAT+ T cells that are present in high abundance in the Peyer’s Patches, supposedly to exhibit both defensive and regulatory roles at the gut mucosal surface, where trillions of microbes reside (Rosas-Ballina et al. 2011). Microbial colonization was shown to be required for the expression of a subset of B cells that expresses acetylcholine receptor (ChAT+B cells), which reside only in mucosal-associated lymphoid tissues (Reardon et al. 2013). Expression of the acetylcholine receptor on ChAT+B cells begins at birth and involves MyD88 dependent toll like receptor signalling. The assumption was evident by the reduction in Ach receptor expression following antibiotic treatment. Taken together, this evolving data strongly supports a key role of the gut microbes in orchestrating psychoneuroimmunology functions.
15.7 From Animals to Humans: Translation of Laboratory Animal Research Evidence
Despite the extensive preclinical data supporting the impact of the gut microbiota on the gut-brain dialogue, limited information is available of how these findings may translate to human in health and disease. A recent study in a healthy cohort of 56 vaginally born Dutch infants was performed to investigate the development of the gut microbiota as a potential pathway linking maternal prenatal stress and infant health (Zijlmans et al. 2015). The findings showed clear links between maternal prenatal stress and the infant gut microbiota. Infants of mothers with high cumulative stress during pregnancy had significantly higher relative abundances of the pathogenic Escherichia, Serratia, and Enterobacter, and lower relative abundances Lactobacillus, Lactoccus, Aerococcus and Bifidobacteria. The disturbed colonization pattern illustrated in this study was related to infant gastrointestinal symptoms and allergic reactions suggesting a role of elevated level of inflammation. Another study conducted in infants with colic demonstrated an overall reduced diversity of the gut microbiota with an increase in Proteobacteria and decrease in Bacteroides in comparison to healthy infants (De Weerth et al. 2013). Although the causal role of microbial dysbiosis in these studies remains elusive, the association between disturbance in microbial composition and clinical phenotypes suggest a potential effect on bacterial interventions on behaviour and health. Indeed, in a placebo-controlled study of healthy women, brain changes in response to a fermented milk product containing four different probiotic interventions were assessed by functional magnetic resonance imaging. This study suggested a basic change in responsiveness to negative emotional stimuli (Tillisch et al. 2013). Brain imaging was also applied in another study conducted in patients with hepatic encephalopathy, mild cognitive disorders, and antibiotic treatment to alter the microbiota composition (Bajaj et al. 2013). Antibiotic treatment resulted in improved cognitive functions and changes in blood fatty acids metabolites that were suggested to be of microbial origin. Even though no changes in the microbial abundance were reported in the study, the results suggested that the improved cognition could be attributed to a shift from pathogenic to beneficial metabolites.
15.8 Conclusions
It is now well established that “healthy” mammalian structure and function is significantly dependent on its residing microbes. Data from animal studies clearly illustrated that unfavourable alterations in the body’s organ systems are linked with microbiota dysbiosis. However, we need a better understanding of the causative mechanisms whereby these interactions occur in order to provide novel avenues to rationally intervene in disease situations with either microbial or dietary interventions that aim to correct imbalance situations and thereby restore homeostasis. To date, limited information is available of how the findings from animal experiments may translate to human in health and disease. The enormous amount of inter-individual variation observed in the microbial composition and its genome require the application of very large studies to distinguish disease-associated changes, which renders the broad application of these trials very complicated within the human population. Moreover, the wide array of variation among individuals in terms of diet, genetics, environmental factors and sex-related differences, adds to the complexity of the human microbiome analysis. Practical and ethical concerns associated with the use of human volunteers represent another challenge that limits clinical intervention studies even with probiotics and if allowed, such studies are generally conducted only in primarily healthy individuals and not the human population that is most at risk.
The expanding repertoire of diseases associated with microbial dysbiosis urges the need to explore what our microbes do and not remain at a stage of describing who they are. Undoubtedly, the major advances in metagenomic and metabolomics technologies are continuing to help the reconstruction of metabolic pathways, which revealed that the microbiome functional diversity is by far less than its genetic diversity, with respect to the inter-individual variation (Human Microbiome Project Consortium 2012). A better understanding of the functionality of the intestinal microbiota will not only help to design future probiotics but also to genetically engineer the microbiome in a way that produces microbial metabolites, which are beneficial to the host or could be applied as nutraceuticals. Moreover, deciphering the dialogue among the microbial community would allow manipulating the community, particularly in susceptible individuals, in a way to remove those bacterial species representing a threat to the balanced microbial consortia, without necessarily being a pathogen.
References
Ader R, Kelley KW (2007) A global view of twenty years of brain, behavior, and immunity. Brain Behav Immun 21:20–22PubMedCentralPubMed
Agans R, Rigsbee L, Kenche H, Michail S, Khamis HJ, Paliy O (2011) Distal gut microbiota of adolescent children is different from that of adults. FEMS Microbiol Ecol 77:404–412PubMedCentralPubMed
Ait-Belgnaoui A, Durand H, Cartier C, Chaumaz G, Eutamene H, Ferrier L, Houdeau E, Fioramonti J, Bueno L, Theodorou V (2012) Prevention of gut leakiness by a probiotic treatment leads to attenuated HPA response to an acute psychological stress in rats. Psychoneuroendocrinology 37:1885–1895PubMed
Al Akeel R (2013) Role of epigenetic reprogramming of host genes in bacterial pathogenesis. Saudi J Biol Sci 20:305–309PubMedCentralPubMed
Alenghat T, Osborne LC, Saenz SA, Kobuley D, Ziegler CG, Mullican SE, Choi I, Grunberg S, Sinha R, Wynosky-Dolfi M, Snyder A, Giacomin PR, Joyce KL, Hoang TB, Bewtra M, Brodsky IE, Sonnenberg GF, Bushman FD, Won KJ, Lazar MA, Artis D (2013) Histone deacetylase 3 coordinates commensal-bacteria-dependent intestinal homeostasis. Nature 504:153–157PubMedCentralPubMed
Alonso C, Guilarte M, Vicario M, Ramos L, Ramadan Z, Antolin M, Martinez C, Rezzi S, Saperas E, Kochhar S, Santos J, Malagelada JR (2008) Maladaptive intestinal epithelial responses to life stress may predispose healthy women to gut mucosal inflammation. Gastroenterology 135:163–172.e161PubMed
Amaral FA, Sachs D, Costa VV, Fagundes CT, Cisalpino D, Cunha TM, Ferreira SH, Cunha FQ, Silva TA, Nicoli JR, Vieira LQ, Souza DG, Teixeira MM (2008) Commensal microbiota is fundamental for the development of inflammatory pain. Proc Natl Acad Sci U S A 105:2193–2197PubMedCentralPubMed
Arumugam M, Raes J, Pelletier E, Le Paslier D, Yamada T, Mende DR, Fernandes GR, Tap J, Bruls T, Batto JM, Bertalan M, Borruel N, Casellas F, Fernandez L, Gautier L, Hansen T, Hattori M, Hayashi T, Kleerebezem M, Kurokawa K, Leclerc M, Levenez F, Manichanh C, Nielsen HB, Nielsen T, Pons N, Poulain J, Qin J, Sicheritz-Ponten T, Tims S, Torrents D, Ugarte E, Zoetendal EG, Wang J, Guarner F, Pedersen O, De Vos WM, Brunak S, Dore J, Meta HITC, Antolin M, Artiguenave F, Blottiere HM, Almeida M, Brechot C, Cara C, Chervaux C, Cultrone A, Delorme C, Denariaz G, Dervyn R, Foerstner KU, Friss C, Van De Guchte M, Guedon E, Haimet F, Huber W, Van Hylckama-Vlieg J, Jamet A, Juste C, Kaci G, Knol J, Lakhdari O, Layec S, Le Roux K, Maguin E, Merieux A, Melo Minardi R, M’rini C, Muller J, Oozeer R, Parkhill J, Renault P, Rescigno M, Sanchez N, Sunagawa S, Torrejon A, Turner K, Vandemeulebrouck G, Varela E, Winogradsky Y, Zeller G, Weissenbach J, Ehrlich SD, Bork P (2011) Enterotypes of the human gut microbiome. Nature 473:174–180PubMedCentralPubMed
Asano Y, Hiramoto T, Nishino R, Aiba Y, Kimura T, Yoshihara K, Koga Y, Sudo N (2012) Critical role of gut microbiota in the production of biologically active, free catecholamines in the gut lumen of mice. Am J Physiol Gastrointest Liver Physiol 303:G1288–G1295PubMed
Bajaj JS (2014) The role of microbiota in hepatic encephalopathy. Gut Microbes 5:397–403PubMedCentralPubMed
Bajaj JS, Hylemon PB, Ridlon JM, Heuman DM, Daita K, White MB, Monteith P, Noble NA, Sikaroodi M, Gillevet PM (2012) Colonic mucosal microbiome differs from stool microbiome in cirrhosis and hepatic encephalopathy and is linked to cognition and inflammation. Am J Physiol Gastrointest Liver Physiol 303:G675–G685PubMedCentralPubMed
Bajaj JS, Heuman DM, Sanyal AJ, Hylemon PB, Sterling RK, Stravitz RT, Fuchs M, Ridlon JM, Daita K, Monteith P, Noble NA, White MB, Fisher A, Sikaroodi M, Rangwala H, Gillevet PM (2013) Modulation of the metabiome by rifaximin in patients with cirrhosis and minimal hepatic encephalopathy. PLoS One 8:e60042PubMedCentralPubMed
Baltimore D, Boldin MP, O’Connell RM, Rao DS, Taganov KD (2008) MicroRNAs: new regulators of immune cell development and function. Nat Immunol 9:839–845PubMed
Bansal T, Alaniz RC, Wood TK, Jayaraman A (2010) The bacterial signal indole increases epithelial-cell tight-junction resistance and attenuates indicators of inflammation. Proc Natl Acad Sci U S A 107:228–233PubMedCentralPubMed
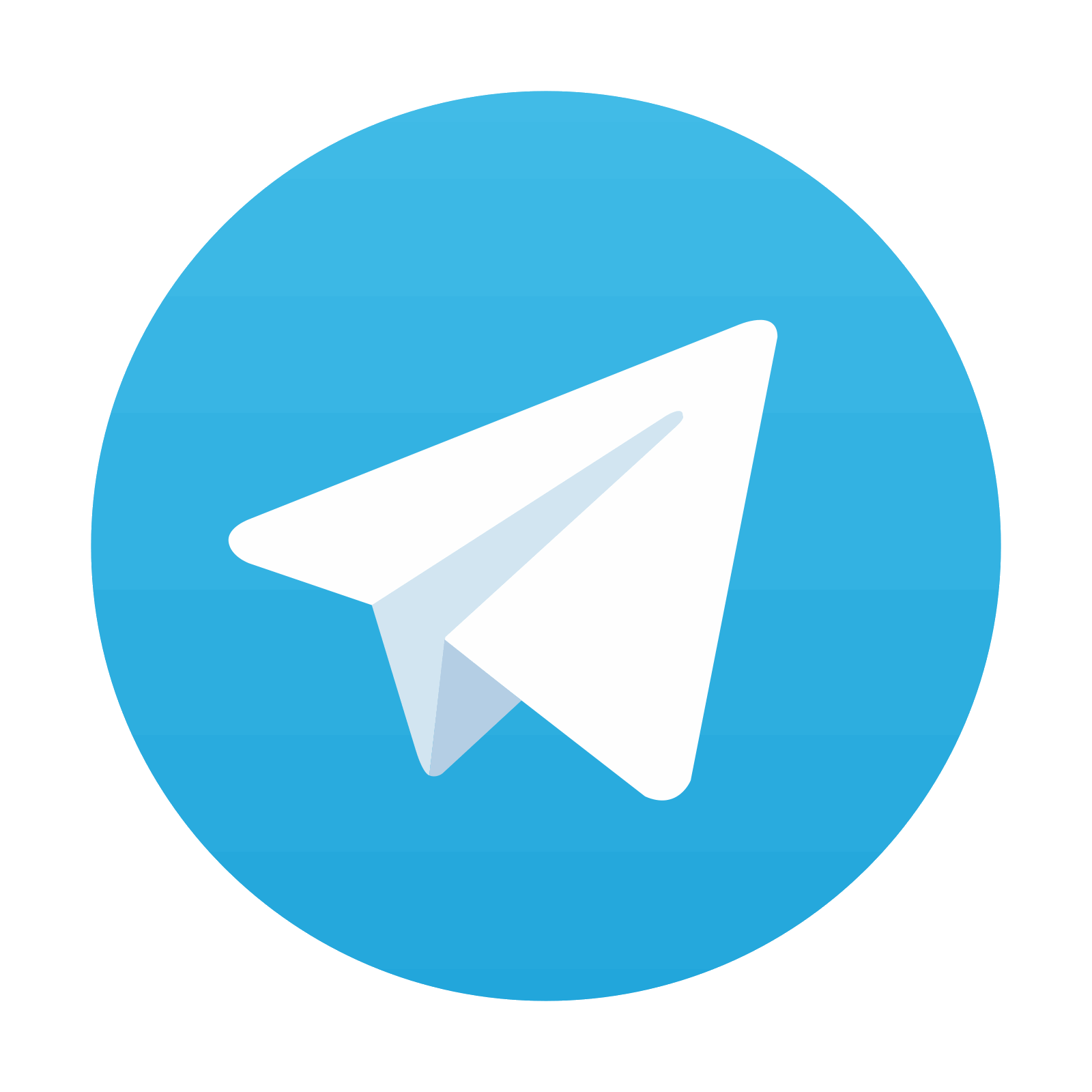
Stay updated, free articles. Join our Telegram channel
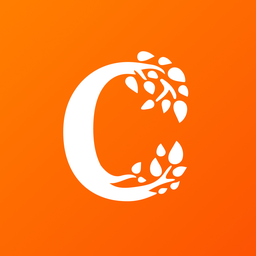
Full access? Get Clinical Tree
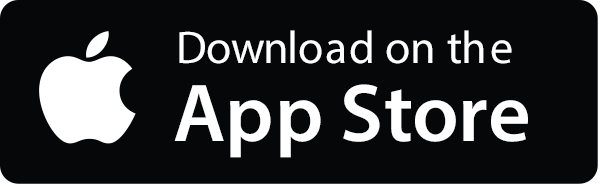
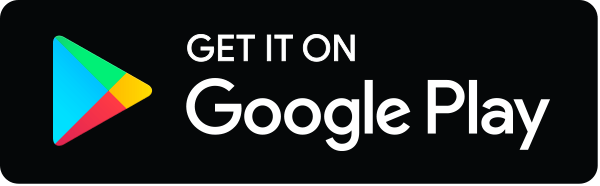