Fig. 1.1
Social conflict in mice is conducted by the simple placement of a group-housed mouse also known as an “intruder” (in picture, black) into the cage of a singly-housed mouse, also known as the “resident” (in picture, white). The resident will engage the intruder ultimately resulting in the “defeat” of the intruder as shown by the limp forepaws and angled ears. Once the intruder assumes the defeat posture, the resident then disengages and at this point the intruder is removed. The social conflict procedure is done under reversed day-night light cycle using low level red light for illumination. For a fuller description of social conflict procedure see Lyte et al. (1990b) and Miczek et al. (2001). The social conflict procedure is done under reversed day-night light cycle using low level red light
However, this surprising result presented a paradox. If immune responsiveness is increased during time of acute, ethologically-relevant stress, then why is the animal more susceptible to an infectious challenge? Most of the literature over the last century had indeed shown that stressed animals did exhibit increased susceptibility to infectious disease challenge (Peterson et al. 1991). With that in mind, I conducted a series of experiments in 1991–1992 in which social conflict stressed animals were challenged with oral pathogens such as Yersinia enterocolitica. The results of those experiments showed the surprising result of increased mortality in stressed animals as compared to home cage controls (Fig. 1.2). Shouldn’t these animals which showed greater than a 500 % increase in phagocytic capacity (Lyte et al. 1990a, b) also display increased resistance to infectious challenge and not the increased mortality (Fig. 1.2)?
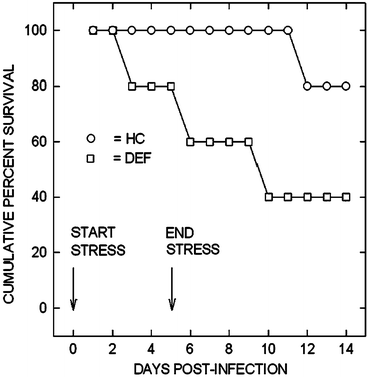
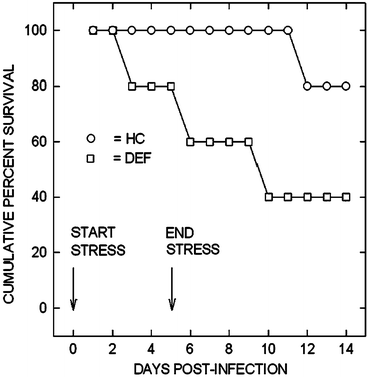
Fig. 1.2
Animals were per orally challenged with Y. enterocolitica immediately prior to social conflict stress (DEF, defeated, squares) or only handling and transport into procedure room (HC, home cage controls, circles). The stress or handling was conducted once per day for 5 days and percent survival followed for 14 days
It was these sets of experiments that in 1991–1992 led me to re-consider the whole concept of stress and susceptibility to infectious disease not from the perspective of the animal, but from that of the infecting bacterium. For a number of reasons, the infecting organism is as highly stressed, if not more so, than the stressed host. First, most infectious agents, such as food-borne pathogens have survived food preservation and cooking steps that result in a damaged cellular state. Upon entrance into the host, the infecting bacterium must survive the host’s physical defenses such as stomach acid and then survive and proliferate within the gastrointestinal tract amid the trillions of indigenous bacteria which rigorously maintain ecological balance among various species through means including, for example, the elaboration of bacteriocins (Riley and Wertz 2002). Central among the factors that influence the ability of any infecting microbe to survive in a host is the capacity to recognize its environment and then employ that information to initiate pathogenic processes (i.e. adherence onto epithelium) and proliferate. The central question then became, what host-derived signals would be available to an infecting bacterium that could be used to the bacterium’s own advantage and ultimately survival within the host? It was at this point that I made the decision to eliminate (for the time being) the role of immunology in addressing the effect of stress on the pathogenesis of infectious disease and instead to concentrate on the role of stress on the infecting bacterium within the hostile environment of the host. In other words, were there direct effects of the host’s stress response on the bacterium?
Critical to the above line of reasoning was an overlooked phenomena of infectious disease as experienced in nature (real-world) as opposed to the laboratory. That aspect specifically concerns the dose of infectious organisms that are needed to effect overt disease in the host. It is well established in food microbiology that the number of infecting organisms needed to cause food-related gastrointestinal infection can be as low as 10 bacteria per gram of food (Willshaw et al. 1994). However, in the laboratory, the challenge of animals with infectious bacteria can well go as high as 1010–11 bacteria or colony forming units (CFU) per mL. Further adding to this discrepancy between real-world and laboratory infectious doses, is that on average a mouse weighs 20–25 g while, on average a human weights 70 kg individual, meaning that the dosage a laboratory animal receives is many-fold greater than what is experienced by an individual. Over the last century a number of investigators have raised the issue of whether non-ecologically relevant doses of infectious organisms can provide complete understanding of the mechanisms that underlie the pathogenesis of infectious disease in vivo (Smith 1996). In a similar fashion, this same question can also be raised regarding in vitro studies which utilize high (>104 CFU per mL) bacterial inoculums. Not unlike the question of how a single individual may respond to a new environment as compared to how a large group of individuals may respond to the same new environment, the survival behavior of low numbers of bacteria within the new environment of the gastrointestinal tract may radically differ from that of large numbers of bacteria. This social aspect of bacterial behavior represents the newly emerging field of sociomicrobiology (Parsek and Greenberg 2005; West et al. 2006). Specifically, the environmental signals that single or low numbers of bacteria may look for markedly differ from that sought by high numbers of bacteria. And in addition to the above point of low, not high, numbers of bacteria which contaminate food, this also applies to the vast majority of infections in general in which infecting doses of bacteria are small (<104 CFU) in number.
Thus, from the outset one of the guiding principles in microbial endocrinology has been the use of low bacterial numbers (1–103 CFU per mL) coupled with a medium that is reflective of the in vivo milieu. Other guiding principles, such as the combination of neuroendocrine hormones and bacteria under study should be matched such that each is found to occur in the same anatomical region in vivo, have also been formulated. In addition to the chapters contained in the present book, the reader is further directed to a comprehensive review which thoroughly discusses the methodological aspects of conducting microbial endocrinology-related experiments (Freestone and Lyte 2008).
The choice of the initial neuroendocrine hormones for the first experiment was based on the stress response itself and the well-known increase in catecholamines (Woolf et al. 1992; Gruchow 1979). Further, the stress-induced release of catecholamines had been one of the primary mechanisms that had been proposed in PNI-related research to account for the ability of stress to suppress immune responsiveness and hence increase susceptibility to an infectious challenge (Ader and Cohen 1993; Webster Marketon and Glaser 2008). As has been recognized for many decades, the induction and sustained release of the catecholamines, especially norepinephrine, occurs during many forms of stress extending from psychological to surgical (Fink 2000). The Gram-negative bacterium, Y. enterocolitica was chosen as the first bacterium to test whether a neuroendocrine hormone, namely norepinephrine, could have direct effects on growth. The results of this initial experiment in 1992, which was carried out in liquid culture using small 60 mm Petri dishes, combined a low inoculum of Y. enterocolitica (33 CFU per mL of serum-supplemented SAPI) with norepinephrine, epinephrine or diluent (Fig. 1.3). In many ways this experiment, which was the proverbial “shot in the dark”, is the one that has led through the many years to the creation of this current book. As shown in Fig. 1.3, there is a very small amount of visual growth evident in both the control and epinephrine supplemented plates (indicated by arrows). However, in the norepinephrine supplemented culture, there is dense growth throughout. To this day I still remember my excitement at seeing these results. And from that day on, I effectively ceased looking at PNI-related phenomena and instead turned my research direction to the study of neuroendocrine-bacterial interactions and the creation of the field of microbial endocrinology.
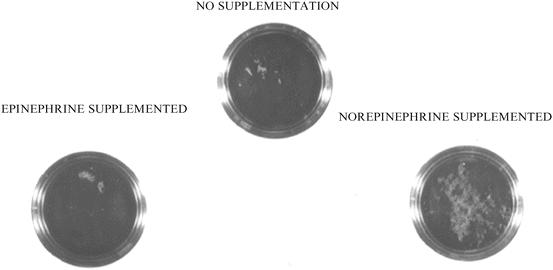
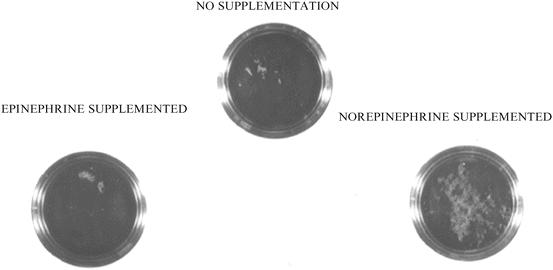
Fig. 1.3
The experiment that launched the field of microbial endocrinology. Yersinia enterocolitica culture plates in 1991 showing that bacterial growth in serum-based medium was enhanced in the presence of the neuroendocrine stress hormone norepinephrine, but not epinephrine or control diluent
1.2.3 Gaining Acceptance of Microbial Endocrinology
As can often be the case in any endeavor which seeks to introduce a paradigm-shift in thinking, the introduction of neuroendocrine-bacterial interactions as a hitherto unrecognized mechanism in the pathogenesis of infectious disease was met not only with initial skepticism, but also downright hostility. At a mid-1990s meeting in Toronto that focused on the role of neuroendocrine mediators and immunity in drug addiction, I gave a microbial endocrinology-based lecture as part of a session on stress and its relationship to drug addiction sequelae such as increased prevalence of infectious disease in drug addicts. At the conclusion of my talk before I could take any questions, the session chair addressed the audience and said that my ideas were so radical that they should not be taken seriously and the audience should in essence forget what I just presented. More than one member of that audience has approached me over the years to recount that episode and the shock of the audience being told to disregard what they had just heard as well to ask why I didn’t get mad (which I didn’t). Such opposition, although admittedly more restrained, was also encountered during the early years in terms of gaining acceptance into the scientific literature. I have been told by more than one individual that the integration of microbial endocrinology into mainstream infectious disease research would have been accelerated if I had chosen to publish in more microbiology-oriented journals. However, my choice to publish in journals not typically read by microbiologists was dictated not by choice, but instead by necessity. My early attempts to publish in basic microbiology-based journals were universally met with rejection. Undoubtedly, while one may take the convenient rode of blaming the reviewer for failure to consider a highly interdisciplinary approach where it is often not possible to address all the questions regarding each of the fields, I shall instead take a fair share of the blame since it is also the responsibility of the author to educate the reader of the need to go beyond traditional thinking.
With that said, I have also come to recognize that one of the defining reasons that these early papers were rejected from basic microbiology-centric journals was the reliance on phenomenology rather than mechanisms. My own training in the clinical laboratory sciences and subsequent work in hospital laboratories before entering graduate school in 1977, ingrained in me a powerful sense of the clinical side of microbiology. And that side is one that is grounded in growth for without evident growth and sufficient numbers of bacteria little can be done, even today, to diagnosis suspected bacterial disease. Thus, it seemed to me at the time (and still does today) that the ability to show growth-related effects of neuroendocrine hormones on bacteria would have profound implications for the study of the host factors which influence susceptibility to infectious disease. However, I was surprised that this was generally not the case. A similar refrain ran through those early reviews that the demonstration of effects on growth were phenomenological in nature and what needed to be shown was the mechanism(s) by which neuroendocrine hormones could influence bacterial physiology. Due largely to the availability of an ever growing arsenal of molecular biological techniques, phenomenology was to be eschewed in favor of dissecting molecular mechanisms. While I do not mean to begrudge nor demean the value of mechanistic studies, one may argue that many of the advances in the treatment of disease have been made through the observation of phenomena for which no mechanism at the time of discovery was available. Antibiotic development owes itself largely to the observation of phenomena. While the requirement for molecular analyses currently reigns dominant in the majority of first-tier microbiology journals, the relegation of phenomenological studies to the status of second-class research ignores its historically pivotal role in fueling scientific and medical advances. A number of articles examining the failure of genomic-based strategies to lead to the discovery of new antimicrobials that ultimately make the transition from the lab bench to clinic have addressed this very point (Finch 2007; Barrett 2005).
My reasoning for discussing the relative merits of phenomenology versus molecular analyses is not to point out my own shortcomings in the area of molecular analysis, but instead to offer a cautionary note to other researchers who may choose to explore microbial endocrinology. Catecholamines, which to date have been the principal neuroendocrine hormones that have been examined in the microbial endocrinology field by virtue of their prominence in the stress response, represent but a tiny sliver of the spectrum of neuroendocrine hormones that can be examined for potential interaction with both pathogenic as well as commensal bacteria. For example, gamma amino butyric acid (GABA), the primary inhibitory neurotransmitter in the mammalian brain, is produced in such large amounts by bacteria in the gut that a role for bacterial-derived GABA has been proposed to account for the altered brain function (encephalopathy) that is part of the pathogenesis of advanced liver disease and sepsis (Minuk 1986; Winder et al. 1988). In fact, GABA produced by bacteria, such as those contaminating a distilled water apparatus, have been found not only to confound neurotransmitter binding studies with mammalian cells (Balcar 1990), but also to possess a high affinity binding protein that resulted in one of the first bioassays for GABA that was entirely bacterial-based (Guthrie and Nicholson-Guthrie 1989; Guthrie et al. 2000). In this book, Chap. 4 by Victoria Roshchina provides an exhaustive review of the wide breadth of neurohormones that are found in prokaryotes that we otherwise only associate with multi-cellular eukaryotic systems.
By utilizing a microbial endocrinology approach, researchers can further our understanding of how host and bacteria, both commensal and pathogenic, interact in the gut (or at other sites). That approach, in turn, could provide insights into not only homeostasis but also other medical conditions that involve gut pathology that upon verification could enable the design of new innovative medical interventions. Although researchers realized more than 100 years ago that the mammalian gut is innervated, how this system interacts with the gut microbial flora remains largely a mystery. Further, large amounts of neurochemicals are produced within the gut that find its way into the gut lumen where the possibility of interactions with the gut microflora exist and remain largely unexplored. For example, large quantities of serotonin are produced by the gut that can be recovered from the lumen, although the physiological reason for this production are not well understood. Could it be that serotonin produced by the mammalian gut has some hitherto unknown interaction with a specific part of microbial population? Thus, examination of any such serotonin-bacterial interaction will depend on both phenomenology and molecular analyses to provide as complete a picture as possible of the relevance of microbial endocrinology to both homeostasis and disease.
Further, the bi-directional nature of bacterial-microbial interactions contained with the theory of microbial endocrinology also suggests that bacteria can influence mammalian function. Work utilizing metabolomics to compare the blood metabolic profile of conventional-reared and germ-free mice revealed that the gut microbiome contributed to the concentration of neuroactive components in the circulation (Wikoff et al. 2009). That the presence of a microbial community within the gut, and inherent interactions between the host and gut microflora, is crucial to an animal’s neurological health was demonstrated in 2004 when Nobuyuki Sudo and colleagues at Kyushu University in Japan examined the role of microbial colonization on the hypothalamic-pituitary-adrenal response to stress in gnotobiotic, germ-free and conventionally-reared mice (Sudo et al. 2004). Not only did the development of host neural systems that control the physiological response to stress depend on postnatal microbial colonization of the gut, but reconstitution of gnotobiotic mice with feces from specific pathogen-free mice altered their subsequent neurohormonal stress response. Additionally, Li et al. demonstrated that diet-induced alteration of gut microbial diversity can even affect memory and learning in mice (Li et al. 2009). Thus we are just beginning to understand the degree to which microbial diversity is crucial to the development and regulation of normal gastrointestinal function. Does gut neuronal activity influence local bacterial ecology and vice versa?
1.3 Collaboration and Dissemination
The development of any field is often dependent on the interactions and potential collaborations with others. Since the initial first sole authored reports in which the concept of direct microbial endocrinology-based interactions (Lyte 1992, 1993) was reported and the theory of its proposed role in health and disease was discussed, it has been the subsequent efforts of graduate students, technicians and fellow scientists that has been instrumental in the growth of microbial endocrinology. While over the course of time some of these collaborations have remained strong, for example, graduate students some of whom later became collaborators in the examination of microbial endocrinology in the microbiota-gut-brain axis (Galley et al. 2014; Lyte et al. 1998), others ended often after running their natural course while others came to a more acrimonious ending. As this chapter is titled, in part, “a personal journey”, the realization that one highly productive collaboration had abruptly ended occurred one late afternoon after a literature search just by happenstance referenced a document in a European data that upon reading, and seeing mutual experimental ideas discussed but no mention that it was indeed collaborative, felt like stepping off a curb, turning to one’s side, and getting hit by a high speed truck. The pursuit of science is not often the straight and collegiate course one imagines as a student.
A critical point in the development of microbial endocrinology turned out to be a fortuitous meeting at the 1995 First International Rushmore Conference on Mechanisms in the Pathogenesis of Enteric Diseases held in Rapid City, South Dakota. Following my presentation, I was approached by a bearded and pony-tailed Richard Haigh, at the time a Ph.D. student at Leicester University in the United Kingdom. Richard’s interest in my work served as the bridge to Primrose Freestone who was a post-doctoral fellow in his lab in the then Department of Microbiology and Immunology within the medical school. During this time I also consider myself fortunate to have helped interest other investigators to examine bacterial-neuroendocrine interactions. For example, during microbiology meetings both in the United States and in Japan at which I and my colleagues had presentations, I was approached by James Kaper of the University of Maryland and his then postdoctoral fellows Jorge Girón and Vanessa Sperandio. Following stimulating conversations with them regarding my concept of bacteria recognizing hormones and the potential of microbial endocrinology, as well as instructions on how to design experiments utilizing neurochemicals, I sent shipments from my lab of neurochemicals to help with initial experiments. Nearly 2 years from the time of my first discussion with James Kaper, Vanessa Sperandio and her colleagues published their landmark paper in PNAS demonstrating the ability of catecholamines to influence quorum sensing in Escherichia coli O157:H7 (Sperandio et al. 2003).
It is axiomatic that the development of any newly emerging field must of necessity rely on the willingness of researchers to freely exchange ideas. Often such exchange is not rewarded with any acknowledgement nor at times proper citation of papers that were instrumental in the founding of the field. As seems to be increasingly the case in science in general, this may be an unfortunate sign of the current times. Regardless, the further theoretical and experimental development of any field must continue to rely on the free exchange of ideas even though the practical aspects of such scientific exchange often do not match the hoped for ideal embodiment of such ideals, especially the regard for proper citation.
Probably one of the greatest fears when one believes to have discovered a new discipline is whether one is really the first. Nearly 5 years following the 1992 publication of my initial papers that helped lay the foundation for microbial endocrinology (Lyte 1992; Lyte and Ernst 1992) I became aware of a reference by the noted physician and essayist Lewis Thomas in his book “The Lives of a Cell: Notes of a Biology Watcher” in which he wrote that one of the forgotten secrets of microbiologists was that the only way to get Clostridium perfringens to establish in mice, in order to evaluate potential antimicrobial agents in the treatment of gas gangrene, was to co-inject epinephrine with the C. perfringens (Thomas 1974). This passing revelation, written by Thomas to illustrate the complexity of biological systems in general, hit me like a thunderbolt that my fear of not properly citing those before me had been realized. What followed turned out to be a journey into a rich history documenting the ability of neuroendocrine hormones to influence the pathogenesis of infectious disease and the many attempts that have been made to understand the phenomena (the majority of these studies have been referenced in my 2004 review, Lyte 2004). In retrospect, given the usage of neuroendocrine hormones such as the catecholamines dating as far back as 1930 in the treatment of clinical conditions ranging from urticaria (itching) (Renaud and Miget 1930) to their current prominent role in the maintenance of cardiac and kidney function in critically-ill patients (Singer 2007), it should not come as that much of a surprise that there has been ample opportunity for the observation of neuroendocrine-bacterial interactions in infectious disease. Indeed, a report detailing the ability of catecholamines to increase aerotolerance of Campylobacter jejuni is instructive of often seminal reports whose significance is not appreciated until many years later (Bowdre et al. 1976; Hoffman et al. 1979). The study of this past literature is highly instructive not simply as only an historical narrative, but as importantly as a guide to future research design from a translational medicine viewpoint since it provides evidence of the role of microbial endocrinology both in health and disease in both animals and humans.
1.4 Microbial Endocrinology and the Microbiota-Gut-Brain Axis
In the first edition of this book, I had offered some future predictions where the field might be heading as well as some cautionary notes (Lyte 2010a). In the intervening 5 years there has been continued growth of the field in the realm of infectious disease as can be well seen in the new and updated chapters in this second edition. At the same time, an increasing number of reports began to appear in the literature suggesting the recognition that the gut microbiota could influence aspects of host behavior (Bercik et al. 2009, 2010; Collins et al. 2013; Neufeld et al. 2011a; Bravo et al. 2011; Cryan and Dinan 2012; Desbonnet et al. 2013; Lyte 2011, 2013b; Bailey et al. 2011). This ability of the microbiota to influence brain function via what has been termed the microbiota-gut-brain axis (Lyte and Cryan 2014), is covered in-depth by the addition of a new chapter to this second edition devoted exclusively to this axis (Chap. 15). The inclusion of this chapter reflects the rapid growth of research into the microbiota-gut-brain axis that was still only nascent at the time of the first edition in 2010 (for comprehensive review see chapters in Lyte and Cryan 2014).
Notwithstanding the recent rapid growth and recognition by many (but certainly not all) in the wider scientific community, as in the case of the initial reports dating back to 1930 of bacteria responding to catecholamines, the proposal that gut microbiota could directly influence brain function has a long history (for comprehensive reviews see Bested et al. 2013a, b, c). The “health” of the colon was seen as paramount and given the bacterial load, as well as the ability at the turn of nineteenth century to culture exclusively aerobic bacteria, it was the obvious anatomical region to link to the brain. Any disturbance in the composition of the colonic contents was linked to alterations in psychological well-being (Bested et al. 2013a). Individuals such as the surgeon H.R. Kellogg (who would later found a cereal company bearing his name to further the idea of colonic health) advocated the removal of the colon itself and its perceived harmful contents, as a way to improve mental health: “Should the colon be sacrificed or reformed … I have labored constantly and earnestly to devise and perfect methods for changing the intestinal flora …” (Kellogg 1917). The inherent difficulty in attempting to manage the composition of the gut microbiota was well-recognized over 100 years ago as it is today: “The control of man’s diet is readily accomplished, but mastery over his intestinal bacterial flora is not … They are the cases that present … malaise, total lack of ambition so that every effort in life is a burden, mental depression often bordering upon melancholia … A battle royal must be fought and when this first great struggle ends in victory for the Bacillus bulgaricus it must be kept on the field of battle forever at guard …” (Stow 1914).
The modern concept of a microbiota-gut-brain axis has relied on an ever-growing number of studies which have demonstrated the ability of bacteria to influence brain function (Bercik et al. 2009, 2010; Collins et al. 2013; Neufeld et al. 2011a; Bravo et al. 2011; Cryan and Dinan 2012; Desbonnet et al. 2013; Lyte 2011, 2013b; Bailey et al. 2011). Initial studies utilized the introduction of novel bacterial species into the gut microbiota that did not cause infection nor activation of the immune system at the doses administered per orally (Lyte et al. 1998). In these studies, the introduction of novel bacterial species such as Campylobacter jejuni into the gut microbiota of mice resulted in activation of specific neural centers in the brain (Gaykema et al. 2004) and that a consequence of this was the induction of anxiety-like behavior (Lyte et al. 1998). The involvement of the vagus nerve, the largest nerve in the body which has numerous branches innervating the gut, in carrying signals from the gut to the brain was demonstrated when partial sub-diaphragmatic vagotomy abrogated the ability of novel bacteria to induce a behavioral response (Goehler et al. 2005). Critical work performed by others, notably the transfer of behavioral phenotype by adoptive transfer of the microbiota has been instrumental in identifying the role of the microbiota-gut-brain axis in behavior. In these experiments performed, the microbiota from a mouse strain displaying an anxious phenotype was adoptively transferred via fecal microbial transplant into another strain which typically exhibited normal, non-anxious, behavior (Collins et al. 2013). The mice which previously displayed only normal behavior, evidenced anxiety-like behavior that was identical to that of the anxious mouse strain from which the fecal microbial transplant was obtained (Collins et al. 2013). The use of germ-free versus conventionally-housed mice has also figured prominently in studies which have examined the ability of the microbiota to influence brain function (Neufeld et al. 2011b; Diaz Heijtz et al. 2011). Indeed the first study to identify the ability of microbiota from specific-pathogen free mice to be adoptively transferred into germ-free mice was that performed by Sudo et al. who showed that following adoptive transfer the hypothalamic-pituitary response to mild restraint stress was attenuated as compared to non-colonized control germ-free animals (Sudo et al. 2004). While such studies involving germ-free animals have been instrumental in furthering the concept of the microbiota-gut-brain axis, a note of caution regarding their use in microbiome-related studies has been raised (Hanage 2014).
Given the capacity of bacteria to produce a wide spectrum of neurochemicals with known ability to affect brain function and behavior, it is therefore reasonable to propose that the in vivo production of neurochemicals by bacteria could influence brain function, and hence behavior, by either of two possible routes. The first would be through the production of neurochemicals that could act locally within the gut by interacting with cognate receptors belonging to the host enteric nervous system. Such interaction would then be communicated to the brain most likely by the vagus nerve. The second route would also involve the production of neurochemicals known to influence behavior, but this route would involve the uptake of the neurochemicals into the portal circulation and its eventual transport into the brain.
With either route, the first question that must be addressed is whether bacteria in the host can produce neurochemicals of sufficient concentration to influence host neurophysiology. Since the publication of the first edition of this book, that question has been answered by Asano and colleagues who published the landmark paper describing the in vivo production of dopamine and norepinephrine by gut bacteria (Asano et al. 2012). In this study, luminal levels of catecholamines in the gastrointestinal tract were measured in specific pathogen-free, germ-free, and gnotobiotic mice. Asano et al. reported that while the catecholamines, norepinephrine and dopamine, were produced in appreciable physiological amounts in the luminal contents of specific pathogen free mice, in germ-free animals substantially lower amounts were detected (Asano et al. 2012). Critically, whereas the majority of catecholamines in pathogen-free animals were structurally determined to be free and biologically active, those found in germ-free animals were present in a biologically inactive, conjugated form. Inoculation of germ-free animals with the flora from specific pathogen free mice resulted in the production of free, biologically active, catecholamines within the gut lumen. As such, this report clearly established that in vivo the microbiota is capable of producing neuroendocrine hormones that are commonly only associated with host production. That these substances also are intimately involved in host neurophysiology provides solid evidence that the fields of microbiology and neurophysiology do intersect with attendant consequences for both host and microbiota as further discussed below regarding the ability of the gut microbiota to influence brain function.
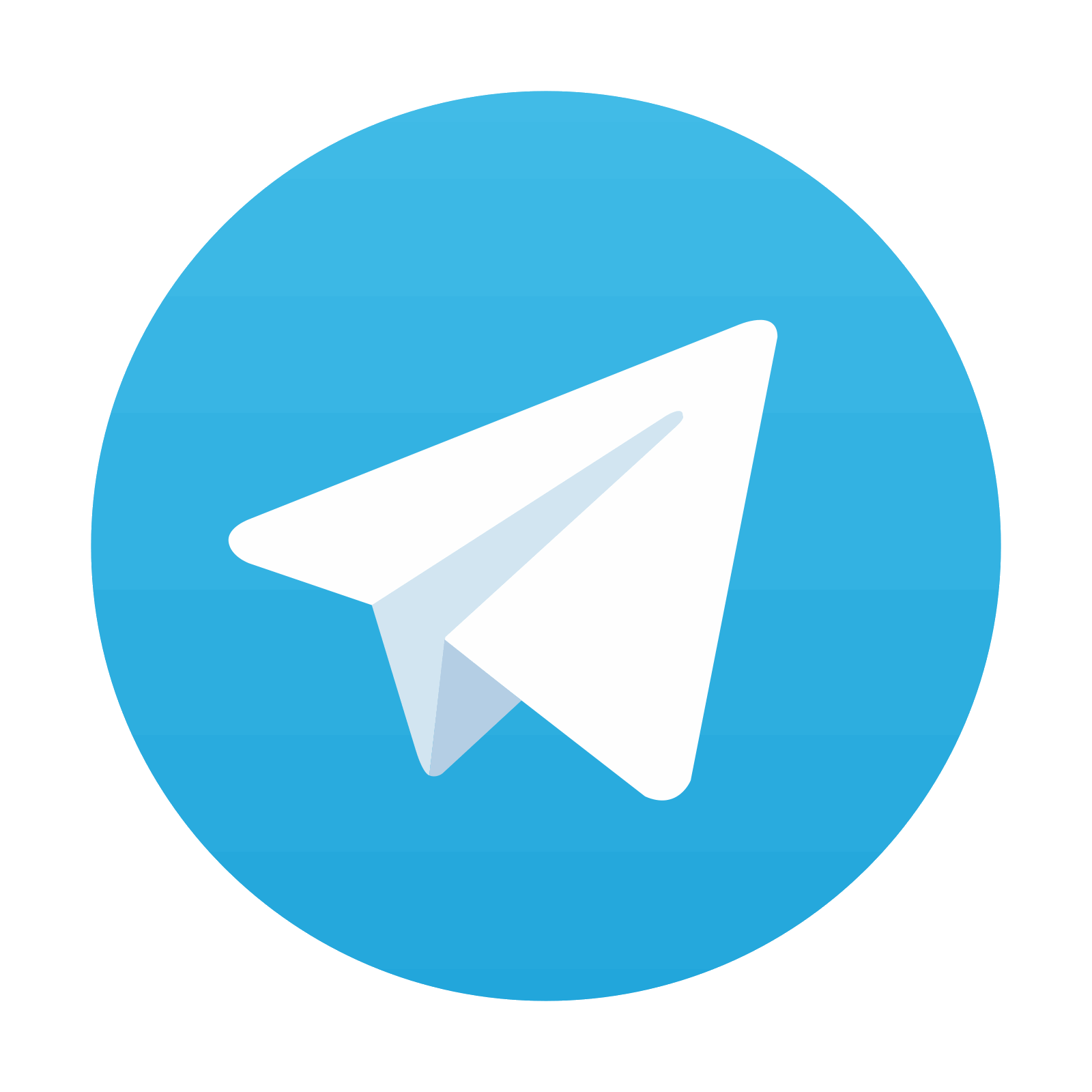
Stay updated, free articles. Join our Telegram channel
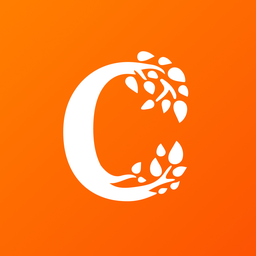
Full access? Get Clinical Tree
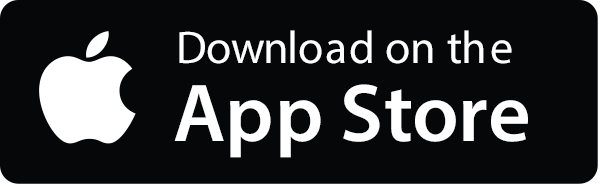
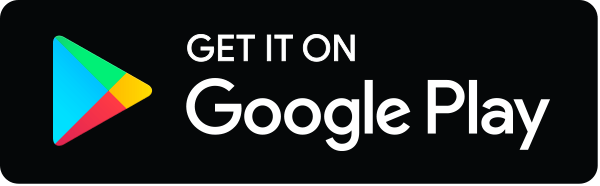