and Nicholas J. Walton1
(1)
Institute of Food Research, Norwich Research Park, Colney, Norwich, NR4 7UA, UK
Abstract
This chapter examines the evidence that the ability of neuroendocrine hormones, notably norepinephrine and epinephrine, to stimulate bacterial growth in iron-restricted media is not limited to molecules with a catecholamine structure but is also possessed by a variety of other catechols, many of which are of plant origin and are common in the diet. Catechols derived from the diet, such as the tea flavanols, can be present in the plasma at submicromolar and micromolar concentrations, comparable with the concentrations of catecholamines that have been shown to be effective in promoting bacterial growth under conditions of iron restriction, although many dietary catechols, notably quercetin derivatives, are present in the plasma and tissues largely as conjugates, from which the catechol function has been lost. Finally, although bacterial growth promotion through relief of iron restriction appears to be exhibited by a wide range of catechols, the gene-activation effects of catecholamines demonstrated to occur in some bacteria may be much more specific, although the definitive experiments to establish structure-function relationships have yet to be reported.
4.1 Introduction
The effects of catecholamines on bacterial growth and behaviour are well documented (see other chapters in this volume) and appear to occur principally through facilitation of iron acquisition from the host glycoproteins, transferrin and lactoferrin. Nevertheless, it is clear that mechanisms other than simple iron acquisition are responsible for some of the effects of catecholamines. The question that naturally arises, however, is how far these phenomena are more generally characteristic of compounds with a catecholic structure, rather than being specific to the neuroendocrine catecholamines, norepinephrine (noradrenaline), epinephrine (adrenaline) and dopamine, or their analogues. This is important, since catechols are common; in particular, they are ingested routinely as components of plant-based diets and often in substantial amounts. A number of subsidiary questions then arise: what are these other catecholic compounds and what are the prevalent dietary sources; what levels of these compounds or of their relevant metabolites might exist in plasma, organs or tissues; and are there any specific effects of catecholamines upon bacteria that are not observed with other catechols, and vice versa? This account attempts to provide some insights.
4.2 Dietary Sources and Distribution of Catechols
Catechols bind iron, and this property is exploited in catecholic siderophores (Crosa and Walsh 2002) and, by contrast, in strategies to prevent bacterial growth by restricting the supply of iron (Scalbert 1991; Mila et al. 1996). Further, since free Fe2+ ions participate in the Fenton reaction with H2O2, which produces highly reactive hydroxyl radicals, the sequestration of free iron by catechols and other iron-chelating compounds can restrict the potential for free-radical generation and protect biological molecules from oxidative damage (Rice-Evans et al. 1995; Khokhar and Apenten 2003). Catechols also possess an autoxidation activity that oxidises Fe2+ to Fe3+, although conversely, reduction of Fe3+ to Fe2+ can also occur, particularly at low pH (Moran et al. 1997; Chvátalová et al. 2008). Catechols with an additional neighbouring hydroxyl group, such as gallic acid (3,4,5-trihydroxybenzoic acid), appear to bind iron and also to oxidise Fe2+ to Fe3+ somewhat less effectively than simple catechols such as protocatechuic acid (3,4-dihydroxybenzoic acid) (Khokhar and Apenten 2003; Andjelković et al. 2006; Chvátalová et al. 2008). As discussed later, a study of the effects of tea catechins revealed that only compounds possessing a 3,4-dihydroxyphenyl group in the B-ring stimulated bacterial growth under iron-restrictive conditions; compounds with an additional neighbouring hydroxyl group in the 5-position failed to stimulate growth (Freestone et al. 2007c). In this chapter, the term catechol is used to denote compounds with the o-dihydroxyphenyl, as distinct from a trihydroxyphenyl, grouping.
Catechols in the diet are most often plant-derived. The large majority are eventual products of the central phenylpropanoid pathway from phenylalanine via cinnamic acid. In plants, phenylpropanoid-pathway derivatives fulfil diverse functions in defence, signalling, protection against ultraviolet light and insect attraction (Parr and Bolwell 2000; Boudet 2007). Those present in the largest amounts are likely to serve a broad-spectrum defence function as anti-feedants or as antimicrobial agents. Catechols are rarely a specific focus of attention, being more often considered nonspecifically within other secondary-product classes, especially the hydroxycinnamic acids and the various sub-classes of the flavonoids. Many plant polyphenolic substances exhibit a broad-spectrum antibacterial activity and catechols are not necessarily among the most potent (Taguri et al. 2006); however, studies of siderophore mutants of Erwinia chrysanthemi demonstrate that plant polyphenols containing catechol groups can act to prevent bacterial growth by sequestering Fe3+, although sequestration of other metal ions, notably Cu2+ and Zn2+, might also occur (Mila et al. 1996). As discussed further, plant catechols include, in particular, catecholic representatives of the benzoic and cinnamic acids and their derivatives, notably protocatechuic acid (3,4-dihydroxybenzoic acid), caffeic acid (3,4-dihydroxy-trans-cinnamic acid) and the chlorogenic acids (principally 5-O-caffeoylquinic acid); oleuropein, a hydroxytyrosol ester found in olives; catecholic flavonols, notably quercetin; catecholic flavanols, for example (−)-epicatechin and (−)-epicatechin gallate; many anthocyanins, which are glycosides of anthocyanidins such as the catechol, cyanidin, and which are widespread as blue, purple and red plant pigments; and finally, many proanthocyanidins or condensed tannins, formally polymerised flavanols. Flavonols, flavones and anthocyanins, though not flavanols, are all generally not found free in plants but are typically found as O-glycosides. Figure 4.1 shows the structures of the principal flavonoid sub-classes.
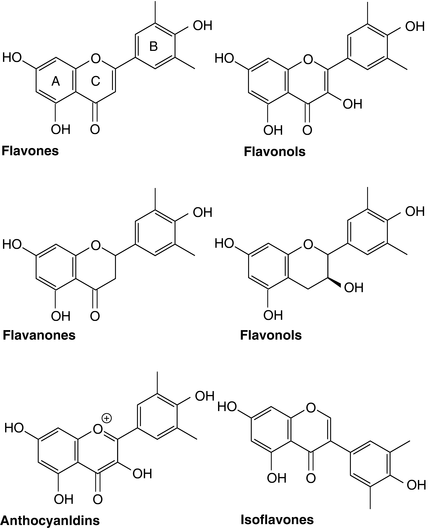
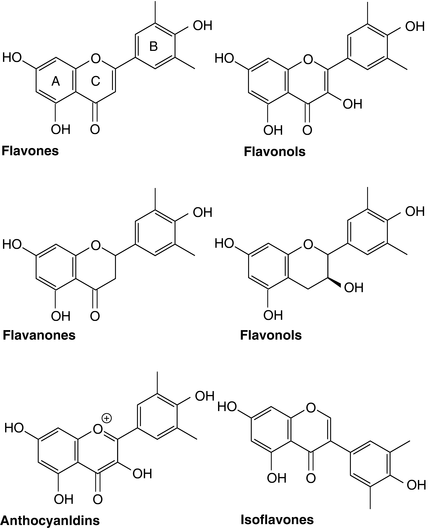
Fig. 4.1
Subclasses of the flavonoids. Classification is based on variations in the heterocyclic C-ring. Reproduced from Hollman and Arts ( 2000), © Society of Chemical Industry, with permission
Many of these compounds attract considerable interest on account of their reported effects in relation to a range of cancers, inflammatory conditions and cardiovascular diseases, which to varying extents (and not in every case) may be a result of their radical-scavenging and antioxidant properties (Ross and Kassum 2002; Cooper et al. 2005; Evans et al. 2006; Hodgson and Croft 2006; Prior et al. 2006; Rahman 2006; Schroeter et al. 2006; Espín et al. 2007; Khan et al. 2008; Loke et al. 2008). One consequence of this is a concern to acquire reliable data on dietary intakes. Extensive information on the contents of phenylpropanoid metabolites in fruits, vegetables and beverages has been compiled (Hollman and Arts 2000; Tomás-Barberán and Clifford 2000; Clifford 2000a, b; Santos-Buelga and Scalbert 2000; Manach et al. 2004). Levels can vary widely on account of varietal characteristics, cultural conditions, developmental stage, position on the plant and storage – and very often within an individual harvested fruit or vegetable (Manach et al. 2004).
Catecholic benzoic and cinnamic acids are amongst the simplest catecholic compounds found in plant foods. They and their derivatives are widespread and may sometimes be present in appreciable amounts. Thus, blackberry fruits may contain ca. 0.07–0.2 g of protocatechuic acid kg−1 fresh weight (Tomás-Barberán and Clifford 2000) and potato tubers may contain around 1.2 g kg−1 fresh weight of chlorogenic acid (principally 5-O-caffeoylquinic acid), though much is likely to be lost during cooking (Clifford 2000a). Coffee also contains large amounts of chlorogenic acid and Clifford ( 2000a) has estimated that 200 ml of instant coffee brew (2% w/v) may provide 50–150 mg of the compound (equivalent to ca. 25–75 mg of caffeic acid).
The occurrence of oleuropein, a secoiridoid glucoside ester of the catechol, hydroxytyrosol, is restricted to olives, where in young fruits it may account for 14% of dry matter, although in processed fruit and in olive oil, levels are lower than this, partly on account of hydrolysis, including hydrolysis to hydroxytyrosol. Olives also contain verbascoside, a compound containing two catecholic residues, hydroxytyrosol and caffeic acid (Soler-Rivas et al. 2000).
In most fruits, vegetables and beverages the levels of flavonols, flavones and flavanols are below about 0.015 g kg−1 fresh weight, although there are conspicuous exceptions (Hollman and Arts 2000). Thus, the catecholic flavonol, quercetin, occurs in the form of glycosides in onions at 0.35 g kg−1 fresh weight and in kale at around 0.11 g kg−1 fresh weight (and in each case possibly considerably more, depending upon variety and cultural conditions); the flavone, luteolin, may reach 0.2 g kg−1 fresh weight in celery leaves; levels of the catecholic flavanols, (−)-epicatechin and (−)-epicatechin gallate, can reach ca. 20–150 µg ml−1 in brewed tea; and levels of (+)-catechin and (−)-epicatechin can reach 100–200 µg ml−1 in some red wines. Cocoa and chocolate are also rich sources of flavanols (Schroeter et al. 2006). Levels of anthocyanins are greatest in those fruits and vegetables that are highly pigmented; for example, blueberries contain ca. 0.8–4.2 g of anthocyanins kg−1 fresh weight and aubergines may contain 7.5 g kg−1 fresh weight (Clifford 2000b). Not surprisingly, red wines, and port wine in particular, contain appreciable levels of anthocyanins, within the range 140–1,100 µg ml−1 (Clifford 2000b). Proanthocyanidins or condensed tannins are abundant in many common or staple foods or beverages (Santos-Buelga and Scalbert 2000), notably black tea, in which two groups of these compounds, the theaflavins and thearubigins, are derived from the flavanols of green tea during processing. Levels of proanthocyanidins of ca. 3–10 g kg−1 dry weight in lentils, of up to 7.4 g kg−1 dry weight in faba beans and of up to 39 g kg−1 dry weight in sorghum, have been determined. High concentrations of proanthocyanidins are often present in red wine and apple juices, and particularly in cider, where levels are reported to range between 2,300 and 3,700 µg ml−1 (Santos-Buelga and Scalbert 2000).
In addition to the catechols discussed above, catecholamines also occur in many plants, and there is evidence for their involvement in defence against pathogens, in responses to plant growth substances and in carbohydrate metabolism, but details of the mechanisms involved still remain uncertain (Kulma and Szopa 2007). Where determined, levels of catecholamines have been found to be low (below 1 mg kg−1 fresh weight), except for bananas, plantains and avocados. Thus, levels in excess of 40 mg kg−1 of dopamine were found in the fruit pulp of red banana and yellow banana, whilst the peel of Cavendish banana contained 100 mg kg−1 (Kulma and Szopa 2007). These values are similar to those of, for example, protocatechuic acid in blackberry fruits (Tomás-Barberán and Clifford 2000). Fruit pulp of Fuerte avocado contained smaller amounts of catecholamines: 4 mg kg−1 of dopamine and <3.5 mg kg−1 of norepinephrine. The relatively high content of dopamine in banana pulp is of particular interest in view of the ability of banana pulp to promote the growth of Gram-negative bacteria in iron-restricted medium, as reported by Lyte ( 1997 ).
Dietary catechols also arise from non-plant sources. In particular, tyramine arises in cheeses and other fermented foods as a result of the bacterial decarboxylation of tyrosine (Santos 1996). Concentrations exceeding 0.1 g kg−1 can be present in matured cheeses (Komprda et al. 2008). Micromolar concentrations of tyramine have been found to increase the adherence of Escherichia coli O157:H7 to murine intestinal mucosa (Lyte 2004b).
4.3 Dietary Intakes of Catechols
Manach et al. ( 2004) reviewed and summarised data from several countries, including the United States, Denmark, the Netherlands and Spain and concluded that the total intake of polyphenols probably reached 1,000 mg d−1 in individuals who ate several portions of fruit and vegetables each day. The consumption of flavonols probably accounted for 20–50 mg d−1, only a proportion of which would be the glycosides of quercetin and other catechols. Levels of anthocyanins consumed were estimated to be broadly similar (though somewhat higher in Finland where appreciable amounts of berries are commonly eaten). However, depending upon individual dietary habits, the intakes of both total and specific polyphenols are likely to be highly variable. In some individuals, chlorogenic acid may predominate as a result of coffee consumption and could amount to more than 200 mg d−1 (Clifford 2000a), whereas drinkers of tea may well ingest comparable amounts of catecholic flavanols and proanthocyanidins (Hollman and Arts 2000; Santos-Buelga and Scalbert 2000; Cooper et al. 2005). Taking all principal sources into account, daily intakes of catechols therefore seem likely to exceed 500 mg d−1 in some individuals.
4.4 Absorption and Availability of Catechols
Although substantial amounts of catechols may be consumed in the diet, many intervening factors and processes will determine whether appreciable concentrations are to be found in the plasma and in tissues. These include the structure of the particular compound, notably the extent and type of glycosylation or esterification; the extent and mechanisms of absorption; the degree of conjugation or derivatisation during or following absorption; potential food-matrix effects; the extent of any metabolism by the gastrointestinal flora, dependent in part upon the degree to which the compound remains unabsorbed (or is possibly re-secreted) in the small intestine; and finally, absorption and metabolism by individual tissues and organs (Manach et al. 2004, 2005; Prior et al. 2006).
Structural effects on absorption are readily apparent, for example, in the case of quercetin glycosides. The 4ʹ-glucoside of quercetin is absorbed more rapidly than the 3β-rutinoside (rutin); maximal absorption of the former occurs about 30–40 min post-ingestion in humans, whereas maximal absorption of the latter requires 6–9 h. Thus, quercetin is found to be absorbed more rapidly from onions, which contain the flavonol predominantly in the form of glucosides, than from apples, which contain it in the form of both glucosides and other glycosides (Hollman et al. 1997). Enzyme systems of the small intestine that can perform the uptake and hydrolysis of quercetin glucosides have been identified and comprise the sodium-dependent glucose transporter, SGLT1, followed by a cytosolic β-glucosidase, or alternatively lactase phorizin hydrolase, a glucosidase of the brush border membrane (Day et al. 2003; Németh et al. 2003; Sesink et al. 2003); glycosides other than glucosides may be absorbed relatively poorly in the small intestine and absorption may be appreciably dependent upon bacterial hydrolysis in the colon. In contrast to flavonols, flavanols are not glycosylated and therefore require no hydrolysis step (Manach et al. 2004). Simple hydroxybenzoic or hydroxycinnamic acids, such as caffeic acid, also appear to be readily absorbed (Clifford 2000a; Cremin et al. 2001), although chlorogenic acid (5-O-caffeoylquinic acid) is not, and hydrolysis by a number of colonic bacteria (strains of E. coli, Bifidobacterium lactis and Lactobacillus gasseri) and by human faecal microbiota has been demonstrated (Couteau et al. 2001; Gonthier et al. 2006 ). Anthocyanins differ from flavonols in that the native glycosides can apparently be absorbed and appear in the plasma, although bioavailability appears comparatively low (Wu et al. 2002; Manach et al. 2005). The major metabolite of cyanidin glucosides in humans is protocatechuic acid (Vitaglione et al. 2007). Proanthocyanidins, particularly those of higher molecular weight, are poorly absorbed, and large proportions are likely to reach the colon (Manach et al. 2004, 2005).
Following absorption, most catechols and polyphenols become derivatised by glucuronidation, sulphation or methylation, or by a combination of these, which are reactions that are undergone by many xenobiotics (Wu et al. 2002; Manach et al. 2004, 2005; Prior et al. 2006). The UDP-glucuronosyltransferase, sulphotransferase and catechol-O-methyltransferase (COMT) activities that are responsible are active in intestinal enterocytes and in the liver; COMT is also active in kidney and has a wide tissue distribution. Prior et al. ( 2006) have summarised the principal patterns of conjugation that are observed for flavonoids. Thus, flavonoids with a catecholic B-ring, such as quercetin and cyanidin, are derivatised mainly to 3ʹ-O-methyl derivatives, with smaller amounts of 4ʹ-O-methyl derivatives. The catecholic B-ring also promotes glucuronidation. For quercetin, the principal compounds detected in human plasma after the ingestion of quercetin glucosides contained in onion were found to be 3ʹ-O-methylquercetin-3-O-glucuronide and quercetin-3ʹ-O-sulphate (Day et al. 2001). Extensive methylation and glucuronidation of the 3ʹ- and 4ʹ-positions also occur in the case of catechin and epicatechin (Natsume et al. 2003).
The bioavailability of polyphenols, including their pharmacokinetics in plasma has been comprehensively reviewed (Manach et al. 2005). For example, meals containing the equivalent of 80–100 mg of quercetin gave rise to plasma concentrations of quercetin conjugates of around 0.3–0.75 μM (Hollman et al. 1997; Manach et al. 1998; Graefe et al. 2001); consumption of 80 g of chocolate (containing a total of 5.3 mg of procyanidins g−1) could produce a plasma epicatechin concentration of as much as 0.36 μM (Rein et al. 2000; Wang et al. 2000); and a glass of red wine containing 35 mg of catechin could produce a plasma catechin concentration of 0.09 μM (Donovan et al. 1999). As reviewed by Manach et al. ( 2004, 2005), half-lives in plasma have been found to be variable, from as little as ca. 2 h for anthocyanins and flavanols to 11–28 h for quercetin derivatives. Therefore, unless dietary catechols are ingested at frequent intervals, their plasma levels, or those of their derivatives, may decline rapidly, and only in the case of compounds such as quercetin glycosides can successive intakes lead to some degree of accumulation of plasma derivatives (Manach et al. 2005). Elimination by both biliary and urinary routes has been demonstrated, with the biliary route likely to be predominant for larger and multiple-conjugated metabolites (Manach et al. 2004).
Besides appearing in plasma, labelled dietary phenolic compounds can give rise to derivatives in a wide range of tissues and organs, especially the digestive organs and the liver. From a number of studies in different laboratories, levels in rats and mice varied between 30 ng and 3,000 ng (as aglycone) g−1 of tissue, depending upon the compound and the dose administered (Manach et al. 2004). It is likely that accumulation in any given organ is non-homogeneous, although it is not clear to what extent organ- or tissue-specific uptake mechanisms may exist.
4.5 Bacterial Growth May be Stimulated Experimentally by a Range of Catechols
It is clear that catechols ingested in the diet, or their derivatives, may be found in plasma and tissues at submicromolar and micromolar levels. These are comparable with, or higher than, normal plasma concentrations of norepinephrine and epinephrine, which are in the nanomolar range (Benedict and Grahame-Smith 1978); and, against this background, we shall shortly examine the evidence from two studies (Coulanges et al. 1998; Freestone et al. 2007c) that have shown that dietary catechols can stimulate bacterial growth in a manner similar to that observed with the neuroendocrine catecholamines, norepinephrine and epinephrine.
However, it is important to keep in mind that a substantial proportion, possibly approaching 50% in some cases (see Prior et al. 2006), of dietary catechol derivatives in the plasma and tissues may be conjugates, in which one of the catecholic hydroxyl groups is methylated, glucuronidated or sulphated. Furthermore, these compounds may not be free in solution. Quercetin incubated with human plasma becomes almost entirely bound to plasma proteins, chiefly albumin (Boulton et al. 1998) and other flavonoids behave similarly, although sulphation and glycosidation may substantially reduce the binding affinity (Dufour and Dangles 2005). The potential effect of protein binding is important because the stimulation of bacterial growth in iron-restricted medium that occurs in response to norepinephrine appears to occur concomitantly with uptake of the catecholamine into the bacterial cell (Freestone et al. 2000). However, the role of norepinephrine uptake in relation to both iron uptake and growth stimulation still remains unclear (Freestone et al. 2003, 2007b). It is not yet known whether the iron uptake and growth stimulation that occur in response to dietary catechols, and which are discussed below, are associated with the bacterial uptake of these compounds. In any event, it remains to be established how far the reported effects of catechols on bacterial growth and behaviour are affected by protein-binding of the catechol. However, the iron-restricted, serum-SAPI medium employed by Freestone et al. ( 2000, 2007c) contains, by definition, the proteins present in adult bovine serum, suggesting that the binding of catechols to proteins is not an issue, at least in this experimental set-up.
In the first of the two studies examining the effects of catechols on bacterial growth, Coulanges et al. ( 1998) examined the growth-promoting effects of a range of catechols upon a number of Listeria species, which (as far as is known) are unable to biosynthesise siderophores. Their ability to overcome growth inhibition induced by the iron-chelator, tropolone, was measured in disk diffusion assays. A number of compounds possessing a catechol grouping were effective in relieving growth inhibition. These included dopamine, epinephrine and norepinephrine and DL-DOPA, the siderophores pyoverdine and rhodotorulic acid, and plant-derived catechols including caffeic acid, esculetin, quercetin and rutin. Salicylic acid (o-hydroxybenzoic acid) was ineffective, as was dihydroxybenzoic acid (the isomer was not specified). This study therefore demonstrated that the relief of tropolone-induced growth inhibition in Listeria monocytogenes that had previously been observed to occur with catecholamines (Coulanges et al. 1997) was not restricted to these compounds but could occur also with a range of nonamine catechols.
In the second study, and following work summarised by Freestone et al. (2002) and Lyte (2004a), Freestone et al. (2007c) examined the growth of E. coli O157:H7 and Salmonella enterica SV Enteriditis in response to a number of catechols commonly consumed in the diet, and to fruit and vegetable extracts known to contain catechols. In the case of the individual catechols, they determined growth responses both in iron-restricted medium and in iron-replete medium and followed up these experiments with measurements of the uptake of 55Fe from 55Fe-labelled transferrin and lactoferrin. Marked differences in growth were observed, depending upon whether a rich medium (Luria broth) or an iron-restricted medium (serum-SAPI) was employed (Figs. 4.2 and 4.3). In the rich medium, none of the compounds tested – catechin, caffeic acid, chlorogenic (5-O-caffeoylquinic) acid and tannic acid – had any significant effect upon growth, with the exception of tannic acid, which became progressively more inhibitory to growth with increasing concentration (up to 200 µg ml−1). In contrast, in the iron-restricted medium (and hence broadly consistent with the findings in Listeria of Coulanges et al. 1998), all four catechol compounds tested promoted growth, by a factor of around 4 log-orders, with saturation occurring at 50–100 µM (50 µg ml−1 for tannic acid). Similarly, all the fruit and vegetable extracts tested (apple, carrot, grape, pear, plum, orange and strawberry), and infusions of tea and coffee, promoted growth in the iron-restricted medium. Study of the effects of tea flavanols (see Fig. 4.4) revealed that the ability to promote growth in the iron-restricted medium was restricted to those compounds possessing a 3,4-dihydroxyphenyl (i.e. catecholic) B-ring ((+)–catechin, (−)-catechin gallate and (−)-epicatechin gallate); trihydroxy-compounds with an additional hydroxyl group in the 5-position of the B-ring ((−)-epigallocatechin, (−)-epigallocatechin gallate and (−)-gallocatechin gallate) failed to promote growth.
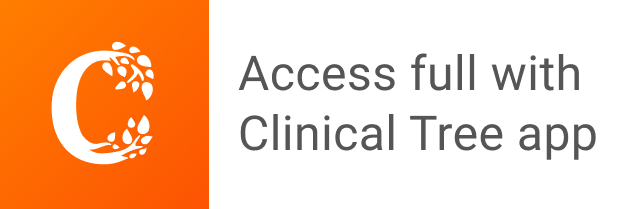