Summary of key facts
- •
Cancer vaccines represent a promising strategy to activate immunity in cold tumors.
- •
The complexity and heterogeneity of human tumors remains a challenge to developing cancer vaccines.
- •
Sipuleucel-T is the first therapeutic vaccine approved for cancer.
- •
Cancer vaccines targeting unmutated tumor-associated antigens (TAAs) have had poor results in the clinic.
- •
Neoantigens that arise from tumor-specific mutations are immunogenic, drive antitumor immune responses, and are promising new are targets for cancer vaccines.
- •
Early results with neoantigen vaccines have shown the most promise in the clinic.
Introduction
Immunotherapy has now emerged as one of the most promising therapeutic approaches to treat cancer. Evidence that the immune system can recognize human cancer and that this recognition can be therapeutically leveraged to control, and even eradicate, widespread cancer has highlighted the power and promise of the immunotherapeutic approach. Antibodies that inhibit immune checkpoint pathway proteins (Programmed Cell Death Protein 1 (PD-1), Cytotoxic T-Lymphocyte Associated Protein 4 (CTLA-4), Lymphocyte Activation Gene 3 (LAG3)) have now demonstrated antitumor efficacy and are U.S. Food and Drug Administration (FDA)-approved for use in melanoma, renal cell carcinoma (RCC), colorectal cancer (CRC), non-small cell lung carcinoma (NSCLC), and Hodgkin lymphoma (HL), among other cancers. Engineered T cells equipped with chimeric antigen receptors (CARs) are FDA-approved for hematologic malignancies including acute lymphoblastic leukemia (ALL), B-cell lymphoma, follicular lymphoma (FL), mantle cell lymphoma, and multiple myeloma. Yet because these approaches undoubtedly represent merely a fraction of the myriad ways through which the immune system controls cancer and could potentially be future therapeutic cudgels, immuno-oncology appears to be in its infancy, with a bright future.
The success of immune checkpoint inhibitors (ICIs) and CAR T cells notwithstanding, what has emerged is that these immunotherapy strategies appear to be successful in only certain tumor types. The impressive results obtained with CAR T cells have appeared restricted to hematologic cancers, and these successes have not so far extended beyond liquid cancers to solid tumors. Similarly, though ICIs have shown broader efficacy across multiple solid and some liquid tumors, their success appears to be mostly restricted to the so-called hot tumors—tumors with greater inherent antigenicity (for instance, due to a higher mutational burden or viral transformation)—which provoke baseline antitumor immune responses that are subsequently disinhibited by ICIs. In fact, ∼80% of cancers appear to be “cold” given lower inherent antigenicity; because they thus provoke weaker baseline antitumor immune responses, they remain insensitive to ICIs.
Thus it is likely that cold tumors, which comprise the majority of current cancers, fail to mount sufficient endogenous antitumor immune responses to render them sensitive to ICIs. , Such cold tumors hence appear halted at an early stage of an immunologic incline ( Fig. 9.1 ) and therefore may require therapies that activate immunity rather than release immune suppression. Cancer vaccines represent one promising strategy to activate immunity.
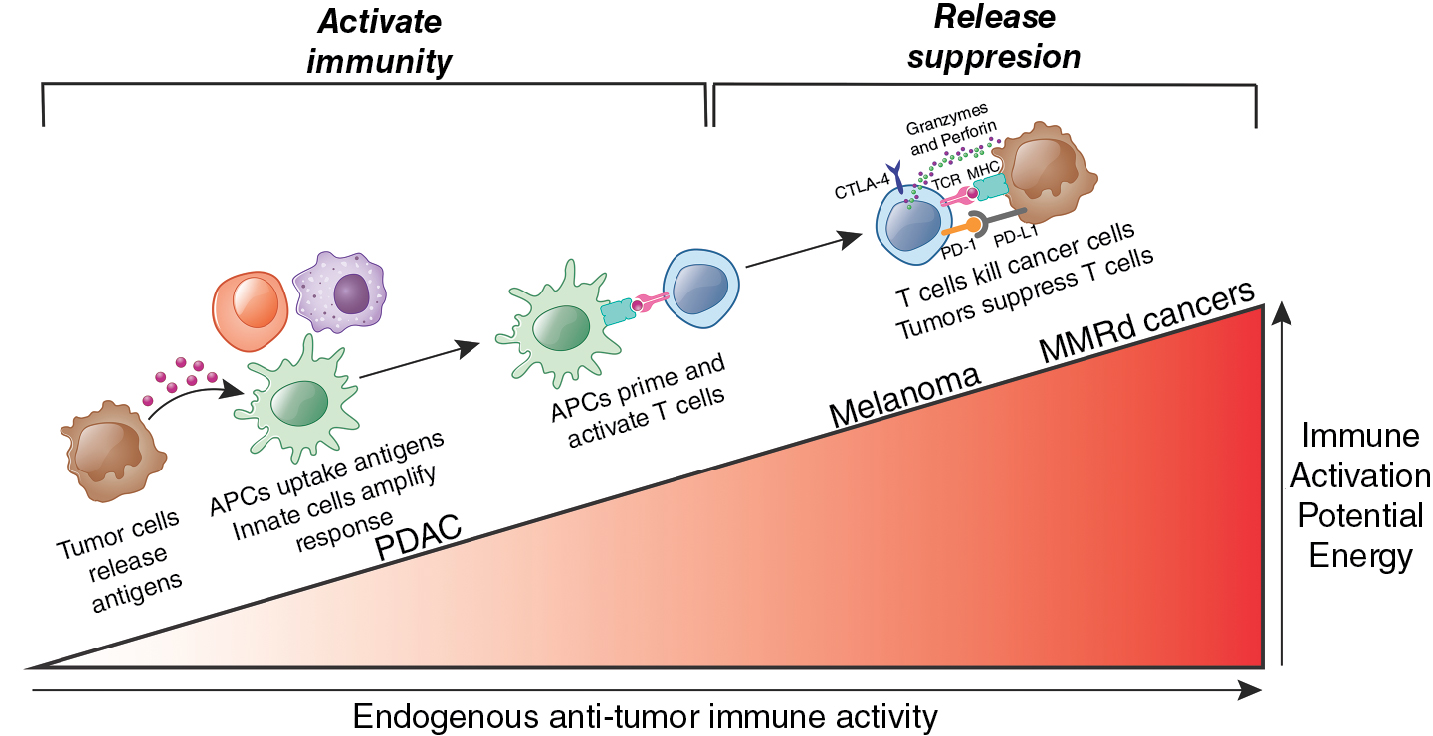
Cancer vaccines
Vaccination is arguably the most important breakthrough in the history of medicine. Vaccines have proven highly effective in preventing infectious diseases caused by viruses or bacteria and have saved hundreds of millions of lives by protecting from, and even eradicating, several deadly infectious diseases such as smallpox, polio, and diphtheria. The success of vaccination stems from its ability to therapeutically harness the inbuilt circuitry of immunologic memory against foreign antigens, namely, that when the human immune system encounters a foreign antigen, it not only rapidly activates immune cells to expand and clear the threat but empowers a subset of these cells to persist long term in the absence of the antigen to reexpand with subsequent exposures. Thus prophylactic exposure to the antigen (i.e., vaccination) activates this circuitry to protect against a future foreign encounter. It remains simple, elegant, and highly effective.
Despite the proof that the immune system protects not only against foreign infectious agents but also oncogenically transformed host cells, unlike vaccination against pathogens, vaccination against host cancer cells has proven significantly more challenging. Though several factors contribute to this challenge, the central hurdle with cancer vaccination has remained the difficulty of choosing optimal tumor antigens for vaccines. Unlike pathogens, tumor cells are intrinsically host cells; thus the majority of proteins in tumors are “self” and hence restricted by central tolerance with their cognate T cells thymically deleted during development. Thus an optimal tumor antigen for a vaccine must be both tumor specific and sufficiently “nonself” to escape central tolerance and allow antigen-specific T cells to escape thymic deletion, enter the peripheral repertoire, and expand upon antigen delivery. Furthermore, given that cancers are genetically heterogeneous collections of transformed cells (more akin to a clade of viruses), further raises the possibility that different oncogenic clones may possess different antigens. These characteristics impose two requirements to identify optimal cancer vaccines: specificity, namely, to discover cancer-specific antigens that are absent from the host proteome during T-cell development, and heterogeneity, namely, to identify cancer antigens that are both sufficiently abundant and antigenic across cancer cell clones and subclones. This necessitates individualized comparisons of tumor and normal host cell genomes and proteomes to identify optimal targets, which has for decades remained technically challenging.
However, breakthroughs in next-generation sequencing technology have made significant advances in this regard—now, comparison of entire genomes of tumor and normal cells is possible. Such analyses have unveiled that most cancers accumulate mutations as they grow, and a fraction of these mutations can generate novel protein sequences completely absent from the human proteome. These “neoantigens” are absent in normal tissues, escape central tolerance to T cells in the thymus, and become T cell targets in cancers. Next-generation sequencing advances have also revealed that T cells recognize neoantigens in cancer (for an in-depth review, see Schumacher et al. ) and induce the success of clinical immunotherapies. Tumors with more immunogenic or “high-quality” neoantigens correlate with greater patient survival , and response with immune checkpoint inhibitors in multiple cancers. Neoantigen-specific T cells induce these clinical responses as well as responses with transfer of autologous tumor-infiltrating T cells. Thus neoantigens have emerged as specific and fundamental immunogenic by-products of cancer pathophysiology, and thus are highly attractive targets for cancer vaccines. Nevertheless, generating effective long-lasting immune responses through vaccination against a tumor has proven much more challenging compared with viruses or other infectious agents because of different factors that will be discussed in subsequent sections.
Antigen choice
A central requirement to develop an effective cancer vaccine is to choose an appropriate antigen. An ideal cancer vaccine antigen would have following ideal characteristics:
- 1.
High immunogenicity (less/not restricted by central tolerance)
- 2.
Tumor specificity
- 3.
Homogeneity across
- (a)
cancer clones
- (b)
patients with cancer.
- (a)
In this regard, tumor antigens can be categorized under two groups: (1) tumor-associated antigens (TAAs) and (2) tumor-specific antigens (TSAs) ( Table 9.1 ).
Antigen Type | Tumor Specificity | Central Tolerance | Shared Among Patients | |
---|---|---|---|---|
Tumor-associated antigens (TAA) | Overexpressed antigens | Low | High | Yes |
Differentiation antigens | Low | High | Yes | |
Cancer/testis antigens | Intermediate | Low | Yes | |
Tumor-specific antigens (TSAs) | Oncogenic viral antigens | High | Absent | Yes |
Public neoantigens | High | Absent | Yes | |
Private neoantigens | High | Absent | No |
Tumor-associated antigens
Tumor-associated antigens (TAAs) are nonmutated self-proteins with preferential or abnormal expression in tumor cells but also some level of expression in normal cells. TAAs can be classified into three main groups:
Overexpressed antigens: This category includes proteins expressed at higher levels in tumors compared with normal tissues. These could be products of genetic amplification or increased transcription, resulting in elevated protein levels. Examples of overexpressed TAAs include human epidermal growth factor receptor 2 (HER-2/neu), human telomerase reverse transcriptase (hTERT), mesothelin, and mucin 1 (MUC-1).
Differentiation antigens: This category includes proteins selectively expressed in differentiated cell types and their derived tumors. Differentiation antigens studied as immunotherapy targets include several in melanomas, including tyrosinase, glycoprotein 100 (gp100), and melanoma antigen recognized by T cells 1 (MART-1), and in prostate cancer, including prostate-specific antigen (PSA) and prostatic acid phosphatase (PAP).
Cancer/testis antigens: This category includes proteins with restricted expression in male germ cells, fetal ovaries, and trophoblasts. Thus these types of antigens are absent in healthy somatic cells but are expressed in a wide variety of tumors. Examples of cancer/testis antigens include the large melanoma-associated antigen (MAGE) family (MAGE-A, MAGE-B, MAGE-C) and New York esophageal squamous cell carcinoma-1 (NY-ESO-1).
Several obstacles limit inclusion of TAAs in cancer vaccines. One of the main disadvantages of TAAs is their low immunogenicity. As outlined previously, TAAs are self-antigens and thus TAA-specific T cells are subject to central tolerance and TAA-specific high-affinity lymphocytes are typically deleted by central or peripheral tolerance. Furthermore, TAA expression is not restricted to tumor cells. These obstacles hamper efforts to use TAAs to induce an immunogenic, tumor-specific immune response.
For overexpressed antigens and differentiation antigens, high levels of protein expression can presumably break tolerance and activate lower affinity lymphocytes. However, they primarily target low-affinity lymphocytes, thus contributing to overall low immunogenicity. The use of highly immunogenic adjuvants and effective costimulators has been proposed to increase the immunogenicity of TAAs. Yet an additional disadvantage remains their lack of tumor specificity and thus the possibility to induce reactivity against corresponding normal tissues and lead to autoimmunity. In support of this, such phenomenon has been observed after adoptive transfer of tumor-reactive T cells directed against overexpressed self-derived differentiation antigens in melanoma. In terms of tumor specificity, cancer/testis antigens theoretically represent a more attractive option because they are not expressed in normal adult tissues but only in germline and embryonic cells. Conversely, one logistic advantage of the use of TAAs for cancer vaccines is that because they are self-proteins and do not arise from patient-specific mutations, they are shared by many patients’ tumors and thus permit universal tumor-specific vaccines rather than personalized vaccines.
Initial clinical trials of cancer vaccines preferentially targeted TAAs because of their easier identification compared with mutation-derived tumor antigens. Preclinical studies have shown that TAAs can be immunogenic in vitro and in murine models in vivo, leading to tumor-protecting immunity. Despite promising results in preclinical studies, results from clinical trials using cancer vaccines targeting TAAs have been in general disappointing. Evidence of TAA-specific CD8 + T-cell responses in vaccinated patients was found, yet clinical efficacy has been rarely reported. The main exception to this would be sipuleucel-T (Provenge, Dendreon Corporation), the first and only therapeutic cancer vaccine approved by the FDA for the treatment of metastatic castration-resistant prostate cancer (mCRPC). Sipuleucel-T is an autologous dendritic cell vaccine prepared by culturing peripheral blood mononuclear cells (PBMCs) from the patient with a recombinant fusion protein of the prostate differentiation antigen PAP and granulocyte-macrophage colony-stimulating factor (GM-CSF), which are then reinfused to the patients. Antigen-presenting cells (APCs) activated by GM-CSF further activate and induce replication of PAP-specific T cells with the capacity to recognize and kill PAP-positive prostate cancer cells. Sipuleucel-T was approved in 2010 based on the results of a phase III trial that showed improved overall survival compared with placebo. Despite their theoretical low immunogenicity, TAAs are still being evaluated as antigens for therapeutic cancer vaccines, and new clinical trials that combine them with other immune checkpoint inhibitors show more promising clinical results and possible correlations with increased survival.
Tumor-specific antigens
Tumor-specific antigens (TSAs) are proteins that are completely absent from normal tissues but only expressed in cancer cells. Thus two obvious advantages over TAAs are that: (1) TSAs are more immunogenic than TAAs because they are not subject to central or peripheral tolerance and thus high-affinity TSA-specific lymphocytes are not eliminated, and (2) TSAs are tumor specific and thus targeting TSAs should less likely induce immune reactivity against healthy tissues and autoimmunity. TSAs can be classified in two groups: (1) oncogenic viral antigens and (2) neoantigens.
Oncogenic viral antigens
Oncogenic viral antigens are viral proteins expressed in cells infected with oncogenic viruses that have subsequently undergone cellular transformation. Viruses can induce cancers, and vaccinating with antigens derived from cancer-inducing viruses is an effective vaccination strategy against such tumors. Because expression of these antigens will be shared among different patients’ tumors, universal vaccines for different tumor types are feasible. Indeed, prophylactic vaccines have been approved to prevent cancers of the cervix, anus, penis, and head and neck induced by human papilloma virus (HPV) and hepatocellular carcinoma induced by the hepatitis B virus (HBV). Both vaccines consist of recombinant virus–like particles (VLPs) that induce immune responses that target the main HPV capsid protein L1 and the HBV surface antigen HBsAg, respectively. However, because human cancers with a known viral etiology are the exception, neoantigens represent the only TSAs targetable for the majority of human tumors.
Neoantigens
Mutations cause cancer and accumulate in cancers as they grow. These mutations can generate novel protein sequences completely absent from the human proteome called neoantigens . These abnormal proteins are degraded in the cytosol by the proteosome to generate short peptides termed neopeptides or neoepitopes . Neoepitopes are then transported to the endoplasmic reticulum, loaded onto major histocompatibility complex (MHC) class I molecules and transported to cell membranes for peptide presentation. Because neopeptides are absent in normal tissues, they escape central tolerance to T cells in the thymus to become T-cell targets in cancers. CD8 + T cells can then recognize the presented neoepitope and acquire cytolytic potential to directly kill tumor cells. Additionally, it is now evident that CD4 + T cells can recognize neoepitopes presented by MHC class II molecules, and their role is also clinically important in controlling tumors in both mice and humans.
Neoantigens are thus both highly immunogenic and tumor specific, which makes them ideal candidates for cancer vaccines. Mounting clinical evidence also indicates that neoantigens are critical antigens targeted by host T cells in human cancers. Advances in next-generation sequencing that now enable neoantigen discovery have revealed that T cells recognize neoantigens in cancer and induce the success of clinical immunotherapies.
Neoantigens are classified based on the type of somatic mutation that alters the protein sequence. The most common somatic mutations that generate neoantigens are as follows:
Single-nucleotide variants (SNVs): Neoantigens are generated by the substitution of an individual nucleotide within an exonic sequence of a gene. Such alterations result in neoantigens with a single amino acid substitution and represent the most common source of neoantigens.
Mutational frameshifts (indels): Single-nucleotide insertions or deletions generate novel amino acid sequence strings because of the shift in the gene reading frame, which may also result in premature stop codons. Such premature stop codons can induce nonsense-mediated decay (NMD) of the translated RNA and prevent protein translation. Nonetheless, indel-derived neoantigens have been correlated with higher immunogenicity and increased clinical response to ICIs.
Fusion genes: Novel amino acid sequences are generated owing to genetic rearrangements that fuse two unrelated genes. Evidence shows that these types of neoantigens can be immunogenic. However, because the frequency of these gene fusions is relatively low, their efficacy as cancer vaccine targets remains unexplored.
Splicing alterations: Mutations can both disrupt and generate de novo splicing sites. Such events can lead to retained introns and alternative exon junctions (“neojunctions”) that generate novel amino acid sequences absent in normal cells that can be presented by MHC molecules. Pharmacologic modulation of RNA splicing has been shown to induce neoantigen generation and to enhance antitumor immunity in mouse models. The use of these types of neoantigens for personalized cancer vaccines has also been proposed, but their efficacy as vaccine targets remains unknown.
The main disadvantage of neoantigens as cancer vaccine antigens is that, because they most frequently arise from stochastic somatic passenger mutations, each tumor has its own profile of neoantigens that is rarely shared among patients. Thus application of neoantigens as a source for vaccine antigens is most common in the context of individualized vaccines, where every patient receives their own private set of mutated epitopes. This is in contrast to TAAs or oncoviral antigens where universal vaccines are feasible. However, advances in next-generation sequencing technologies and bioinformatics now allow for rapid identification of candidate neoantigens on a per patient basis.
Although the vast majority of neoantigens arise in passenger genes and are thus private, public neoantigens arising from driver genes are also possible therapeutic targets. Theoretically, targeting public neoantigens for therapy offers advantages over targeting private neoantigens. First, it avoids many of the challenges of developing personalized vaccines. Manufacturing a therapeutic vaccine de novo for each patient requires complex logistics and higher costs, which have in general raised doubts about the widespread accessibility and feasibility of the personalized approach. Additionally, the extended timeline of design and manufacture increases the risk of disease progression before treatment can be initiated. Second, because driver gene mutations drive tumorigenesis and are indispensable for tumor growth, they should be present in most cells within the tumor. Furthermore, because losing the mutation would result in a loss of tumor fitness, tumor cells are less likely to acquire therapy resistance by means of neoantigen loss. This is in contrast to passenger neoantigens, which are subclonal, do not usually provide an advantage in tumor fitness, and thus are more likely to be lost during clonal evolution and induce therapy resistance. Despite these theoretical advantages, public neoantigens also present some important limitations that hinder their applicability as off-the-shelf therapeutic cancer vaccines. One potential problem is that most patients have just one or very few public neoantigens in their tumors, in contrast to private neoantigens, which are present at a much higher multiplicity. Multiepitope vaccines are thus less feasible when targeting neoantigens in driver genes, which increases the risk of therapy resistance. Another important barrier of public neoantigen therapy is that most driver mutations appear to not be immunogenic, with immunogenic driver mutations requiring the presence of rare human leukocyte antigen (HLA) alleles. Thus for a public neoantigen-targeted therapy to be applicable to different patients, it needs to share not only the specific driver mutations but also the specific HLA alleles that can allow the neopeptide to be presented as immunogenic. Considering frequencies of the most common HLA alleles and most common driver mutations, it was calculated that patients with pancreatic cancer that could potentially benefit from anti-KRAS G12D therapy is around 10%. If a collection of public neoantigen-targeted therapies is developed, they could potentially treat millions of patients with cancer. Yet personalized approaches will still be necessary for the vast majority of cases.
Neoantigens are currently conceived as the most promising antigens in cancer to induce antitumor immune responses. Several studies have shown that higher neoantigen burden is associated with increased baseline immune cell tumor infiltration and T-cell activation and improved clinical outcome in patients with different types of cancer. , Clinical response to ICIs has also been associated with mutations and thus neoantigen load in patients with different tumors including melanoma, NSCLC, and CRC with mismatch repair deficiency. Furthermore, expansion of neoantigen-specific T cells was found in patients showing clinical responses to anti-CTLA4 , and anti-PD-1 treatment. However, tumor mutational burden and neoantigen load do not always predicts immunotherapy response, suggesting that there might be other immunologic factors that predict neoantigen immunogenicity. For example, in patients with pancreatic cancer or glioblastoma, survival was not correlated with neoantigen quantity but with neoantigen quality (see Nonselfness of microbial vs tumor antigens ). In any case, this suggests that even in cancer with low mutational burden and baseline T-cell activation (cold cancers), tumor neoantigens might drive antitumor immune responses and play a key role in patient survival. , Overall, it has now been demonstrated through mouse models and human studies that neoantigens elicit cytolytic responses that contribute to tumor regression and that neoantigens are key targets of the most successful immunotherapies including immune checkpoint blockade and adoptive cell transfer.
Cancer vs pathogen vaccines
Prophylactic vs therapeutic approach
Infectious diseases are caused by specific microbes. Developing a vaccine against such diseases requires previous knowledge of the etiologic agent, its genome, proteome, and therefore the potential immunogenic antigens. Hence, barring putative mutations that could lead to antigenically distinct variants, the antigens that could elicit a protective immune response will be common among the pathogen and thus shared among all infected individuals. Thus a universal vaccine that contains such antigens can be prophylactically administered to healthy individuals who are yet to contract the disease. This can lead to immunologic memory that can prevent future infection and therefore pathogen spread. In the case of a new disease like the coronavirus disease 2019 (COVID-19) pandemic, modern technologies have allowed for an exceptionally rapid identification of the virus, its genome, and the lightspeed development of several effective vaccines. Since the first cases were reported in China, the first severe acute respiratory syndrome coronavirus 2 (SARS-CoV-2) genome sequence was released approximately 1 month after, the first phase I clinical trials started 3 months after, and the FDA approved the first two vaccines 1 year after. This unprecedented speed of vaccine development supports vaccination as the most effective approach to fight infectious diseases.
This is in contrast to tumors, which rarely have universal antigens. Genome instability and accumulation of mutations are a hallmark of cancer, and during tumor evolution most genetic mutations are acquired stochastically. As a result, each person’s cancer has a unique combination of genetic alterations, and even different cells within the same tumor harbor different mutations. This high antigenic variability adds significant complexity to developing universal cancer vaccines and thus hinders the feasibility to treat cancer prophylactically.
An exception to this is infection-associated cancers. In 2018, approximately 13% of all cancers diagnosed worldwide were associated with infection. Although the bacteria Helicobacter pylori is responsible for most cases, viruses represent the most common etiologic agent overall. At least eight viruses have been identified to cause cancer, HPV, HBV, hepatitis C virus (HCV), Epstein-Barr virus (EBV), Kaposi sarcoma–associated herpesvirus (KSHV or HHV-8), Merkel cell polyomavirus (MCV), human T-lymphotropic virus type-1 (HTLV-1), and human immunodeficiency virus (HIV). These viruses can induce oncogenesis either directly through expression of viral oncoproteins (HPV, HTLV-1), or indirectly by inducing chronic inflammation (HBV or HCV) or compromising the immune system and the immunosurveillance of malignant cells (HIV). The theoretical advantage of virus-induced tumors is that vaccines can be developed to target the viral antigens and therefore can be used prophylactically. Indeed, prophylactic vaccines against two virus-inducing tumors have been approved by the FDA, including vaccines against HPV (associated with cancers of the cervix, anus, penis, and head and neck) and HBV (associated with hepatocellular carcinoma [HCC]). The HBV vaccine can be considered the first FDA-approved vaccine to prevent cancer. The first version approved in 1981 was a blood-derived product that was replaced in 1986 by a safer recombinant virus–like particle (VLP) vaccine. Twenty years later, in 2006, the FDA approved the first HPV vaccine, also a VLP-based vaccine. Overall, three vaccines have been approved conferring protection against different HPV subtypes: Gardasil (Merck) against subtypes 6, 11, 16, 18; Cervarix (GlaxoSmithKline) against subtypes 16 and 18; and Gardasil 9 (Merck) against subtypes 6, 11, 16, 18, 31, 33, 45, 52, and 58.
Because preventing virus infection is an effective mechanism to prevent virally induced cancers, the efficacy of these vaccines is mostly attributed to the induction of virus-neutralizing antibodies. Hence, although these vaccines work prophylactically, they have limited therapeutic efficacy for patients with already established virus-induced tumors, where T-cell responses are more desirable. In the case of HPV, tumor progression is often associated with the lack of virus-specific T-cell immunity or the presence of dysfunctional and suppressive HPV-specific T cells.
Looking more broadly, despite these advances, human cancers with a known viral etiology are a minority. Vaccination approaches for the remaining 87% of human cancers will require the use of tumor antigens arising from the patient’s own genome, impeding the prophylactic approach.
Nonselfness of microbial vs tumor antigens
Arguably, one primary function of the immune system is to protect the host from pathogens. For that purpose, the peripheral T-cell repertoire is pruned to recognize non-self-antigens and, to avoid autoimmunity, thymic selection deletes T cells with high affinity to antigens derived from the human proteome. Therefore our peripheral lymphocyte repertoire has been shaped to avoid recognition of self-antigens. In theory, this poses a great challenge to vaccination against cancer because unlike microbial pathogens, tumor cells are in principle self. This lack of immunogenicity against self-proteins is likely one of the key factors why cancer vaccines using unmutated tumor-associated antigens (TAAs) have in general been ineffective.
Nevertheless, it is now widely accepted that another key function of the immune system is to surveil for aberrant cells that could potentially lead to cancer. Although this “cancer immunosurveillance” theory was proposed in 1957, newer studies from 2002 have strengthened this concept, demonstrating that the immune system not only eliminates nascent transformed cells but also sculpts developing tumors. Multiple studies have demonstrated that the relevant targets of the immune system in such transformed cells do not seem to be unmutated TAAs but rather neoantigens that arise as a result of somatic mutations in the cancer cell genome. Theoretically, because neoantigens are absent in normal cells, putative T cells recognizing them are not deleted during thymic selection and populate the peripheral repertoire. As in the case of microbial antigens, this bypass of central tolerance would render them as non-self-antigens. Indeed, neoantigens have been shown to induce protective responses against cancers and are targets of adoptive T-cell transfers or immune checkpoint therapies. , , But are neoantigens as nonself as microbial antigens? In reality, a large fraction of computationally predicted neoantigens fail to induce detectable immune responses. This could be attributable to different factors, such as inaccurate in silico predictions, immunodominance, or the suppressive microenvironment of a tumor. However, another possibility is that many neoantigens might still fall within the self-peptide space. Although neoantigens can arise from different types of mutations (see Neoantigens ), efforts have been especially focused on nonsynonymous SNVs, mainly because they are the most common type in most cancers. SNV neoantigens differ only by one amino acid from the wild-type self-peptide. However, some of these amino acid changes might generate neopeptides that are too antigenically similar to self and thus not sufficiently immunogenic to trigger an immune response.
Mechanisms underlying neoantigen immunogenicity are still not fully understood, which creates difficulty in rational design of neoantigen vaccines. Dissimilarity to the self-proteome has been proposed as a predictor of peptide immunogenicity. If an amino acid substitution results in a presented neopeptide with a high degree of similarity to the self-antigen, namely, three-dimensional shape or T-cell receptor (TCR) contacts, one would think that putative self-antigen-specific T cells that could cross-react against the neoantigen would have been deleted by thymic selection.
Interestingly, another possible theory postulates that neoantigens with high similarity to non-self-peptides, namely microbial antigens, may possess higher immunogenic potential. , , The most accepted mechanism for this theory is molecular mimicry, where lymphocyte receptors with specificity to microbial epitopes could potentially cross-react against highly homologous neoantigens. This phenomenon of cross-reactivity has been widely documented in the context of autoimmune diseases and has gained more strength in the last few years in the context of cancer. Snyder et al. showed that tumors from patients who exhibited long-term clinical benefit from CTLA-4 inhibition contained immunogenic neoantigens that shared sequences that were homologous to many viral and bacterial antigens from the Immune Epitope Database (IEDB). Such a sequence signature was absent in patients with minimal or no benefit from the immune checkpoint treatment. Furthermore, this neoantigen signature showed stronger correlation with survival than did mutational load. Additional support for the clinical relevance of the mimicry theory was shown with the development of a neoantigen quality fitness model. This model, which confers a higher immunogenicity score to neoantigens with differential MHC presentation and homology to infectious disease–derived peptides, predicted survival in two independent cohorts of immunotherapy-naïve patients with pancreatic cancer based on neoantigen quality. Moreover, T-cell clones with specificity to both high-quality neoantigens and predicted cross-reactive microbial peptides were found to infiltrate the tumors of long-term survivors of pancreatic cancer. The same model also predicted survival in anti-CTLA-4-treated patients with melanoma and anti-PD-1-treated patients with lung cancer. Subsequent studies further confirmed the predictive power of the quality model in glioblastoma. In the aforementioned cohorts, neoantigen quality was a better predictor for survival than neoantigen burden. Subsequent improvements to this model to rationally select immunogenic neoantigens based on immunogenic features of “high quality” have identified immunogenic neoantigens naturally targeted by the endogenous repertoire in cancers and longitudinally edited. 43
In addition to pathogenic microbes, the commensal microbiota has been described to play an important role in molecular mimicry. Theoretically, the gastrointestinal tract is considered a tolerogenic environment to avoid immune rejection of commensal gut microbiota. Thus it would be logical to posit that neoantigen homology to epitopes of commensal microbiota would be tolerated and lowly immunogenic. However, evidence seems to point otherwise. Indeed, it has been proven in different mouse models that having a specific bacterial strain in their gut microbiota confers better tumor protection and that such protection was mediated by cross-reactive microbiota-specific T cells. , Similarly, the gut microbiome also seems to play an important role in responsiveness to immune checkpoint blockade. Bifidobacterium was found to be associated with the antitumor effects of anti-PD-L1 therapy, whereas Bacteroides thetaiotaomicron or Bacteroides fragilis was associated with response to CTLA-4 blockade. The latter association was also found in patients with cancer in whom T-cell responses specific for such bacterial strains correlated with improved efficacy of CTLA-4 blockade.
The likely explanation for this phenomenon remains skewing of the peripheral T-cell repertoire toward recognizing common immunogenic features in antigenic peptides, which is then reflected as cross-reactivity of T cells between peptides that harbor such features (e.g., microbial peptide and neopeptide). One interesting therapeutic potential of this cross-reactivity is that heteroclytic vaccination with microbial peptides could elicit potential cross-reactive protection against homologous tumor antigens. These concepts remain areas of active investigation and will be critical to elucidate the features that distinguish immunogenic and nonimmunogenic neoantigens.
Antigen delivery systems
Human tumors are complex, with varying antigens and levels of expression. For this reason, efficient cancer vaccination platforms should ideally capture the breadth of the tumor antigenic space and theoretically include vaccines with multiple tumor antigens to prevent outgrowth of antigen-negative tumor cells. To achieve this, different vaccination platforms have been tested in the clinic. Among those, cell-based vaccines, peptide vaccines, and mRNA vaccines are the most advanced. These strategies can either be antigen agnostic, where whole tumor cells or tumor cell lysates are used as an antigen source without selection, or antigen specific, where prior antigen identification and selection is performed. The characteristics of these vaccine platforms and their application in the clinic are discussed later ( Table 9.2 ).
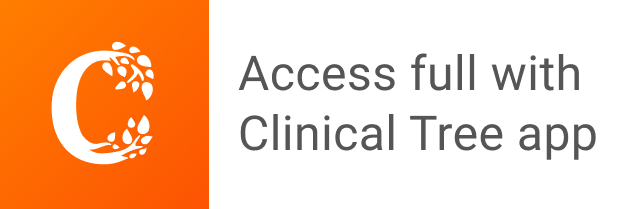