INTRODUCTION
SUMMARY
The marrow, located in the medullary cavity of bone, is the site of hematopoiesis in humans.* The marrow produces approximately 6 billion cells per kilogram of body weight per day. Hematopoietically active (red) marrow regresses after birth until late adolescence, after which it is focused in the lower skull, vertebrae, shoulder and pelvic girdles, ribs, and sternum. Fat cells (yellow marrow) replace hematopoietic cells in the bones of the hands, feet, legs, and arms. Fat comprises approximately 50 percent of red marrow in the adult. Further fatty replacement of the red marrow continues slowly with aging, but hematopoiesis can be expanded when demand for blood cells is increased.
The marrow stroma consists principally of a network of sinuses that originate at the endosteum from cortical capillaries and terminate in collecting vessels that enter the systemic venous circulation. The trilaminar sinus wall is composed of endothelial cells; a thin basement membrane; and adventitial reticular cells that are progenitors of chondrocytes, osteoblasts and adipocytes. Stem cells can leave and reenter marrow as part of their normal circulation.
Hematopoiesis, the proliferation and differentiation of stem cells and their progeny in the intersinus spaces, is controlled by a complex array of stimulatory and inhibitory cytokines, cell–cell contacts, and interactions with the extracellular matrix. In this unique environment, lymphohematopoietic stem cells differentiate into all the blood cell lineages. Mature cells are produced and released to maintain steady-state blood cell levels. The hematopoietic system also can respond to meet increased demands for additional cells as a result of blood loss, hemolysis, inflammation, immune cytopenias, and other causes.
Acronyms and Abbreviations:
AGM, aorta-gonad-mesonephros; ALCAM, activated leukocyte adhesion molecule; bFGF, basic fibroblast growth factor; BFU-E, burst-forming unit–erythroid; BMP, bone morphogenetic protein; CAR, CXCL 12–abundant reticular cells; CD, cluster of differentiation; C/EBP, CCAAT/enhancer-binding protein; CFU-E, colony forming unit–erythroid; CFC-G, colony-forming cell-granulocyte; CXCL12/SDF1, stromal cell-derived factor; dpc, days postcoitum; EBI, erythroblastic island; ECM, extracellular matrix; ELAM, endothelial leukocyte adhesion molecule; EPO, erythropoietin; FN, fibronectin; GAG, glycosaminoglycan; G-CSF, granulocyte colony-stimulating factor; GM-CSF, granulocyte-macrophage colony-stimulating factor; GMP, granulocyte-macrophage progenitor; HGF, hepatocyte growth factor; HIF, hypoxia-inducible factor; HSC, pluripotent hematopoietic stem cell; ICAM, intercellular adhesion molecule; IHH, Indian hedgehog family of proteins; IL, interleukin; LFA, lymphocyte function antigen; MAdCAM, mucosal addressin cell adhesion molecule; M-CSF, macrophage colony-stimulating factor; MEP, megakaryocytic-erythroid progenitor; MIP, macrophage inflammatory protein; MMP, matrix metalloproteinase; MPP, multipotential pluripotential progenitor; MSC, mesenchymal stem cell; NFAT, nuclear factor of activated T cells; NK, natural killer; OPG, osteoprotegerin; PDGF, platelet-derived growth factor; PECAM, platelet endothelial cell adhesion molecule; PPAR, peroxisome proliferator-activated receptor; ProEBs, proerythroblasts; PSGL, P-selectin glycoprotein ligand; RANK, receptor activator of nuclear factor-κB; Rb, retinoblastoma tumor-suppressor protein; SCF, stem cell factor; Siglecs, sialic acid-binding immunoglobulin (Ig)-like lectins; SP, side population; TGF-β, transforming growth factor-β; TLR, toll-like receptor; TNF-α, tumor necrosis factor-α; TPO, thrombopoietin; TRAP, tartrate-resistant acid phosphatase; TSP, thrombospondin; VCAM, vascular cell adhesion molecule; VEGF, vascular endothelial growth factor; VLA, very-late antigen.
*Marshall A. Lichtman was an author of this chapter in the previous six editions, and some material from those editions, including all illustrations, has been retained.
HISTORY AND GENERAL CONSIDERATIONS
The marrow, one of the largest organs in the human body, is the principal site for blood cell formation. In the normal adult, daily marrow production amounts to approximately 2.5 billion red cells, 2.5 billion platelets, and 1 billion granulocytes per kilogram of body weight. The rate of production adjusts to actual needs and can vary from a basal rate to several times normal. Until the late 19th century, blood cell formation was thought to be the prerogative of the lymph nodes or the liver and spleen. In 1868, Neuman1 and Bizzozero2 independently observed nucleated blood cells in material squeezed from the ribs of human cadavers and proposed that the marrow is the major source of blood cells.3 The first in vivo marrow biopsy probably was done in 1876 by Mosler,4 who used a wood drill to obtain marrow particles from a patient with leukemia. Studies by Arinkin5 in 1929 established marrow aspiration as a safe, easy, and useful technique (Chap. 3).
Kinetic studies of marrow cells, using radioisotopes and in vitro cultures, have shown that cell lineages consist mainly of maturing cells with a finite functional life span. On the other hand, sustained cellular production depends on pools of primordial cells capable of both differentiation and self-replication.6 The most primitive pool consists of pluripotential lymphohematopoietic stem cells with the capacity for continuous self-renewal, that is, hematopoietic stem cells (HSCs). The more mature pools consist of differentiating progenitor cells, with their maturation restricted to single or limited numbers of cell lineages and more restricted capacities for self-renewal (Chap. 18). The proliferative activity of these pools involves humoral feedback from peripheral target tissues7 and cell–cell and cell–matrix interactions within the microenvironment of the marrow.8 The marrow stroma and nearby hematopoietic cells provide unique structural and chemical environments (niches) that support the survival, differentiation, and proliferation of pluripotential HSCs. HSC interactive niches9 have been identified at the structural and molecular10 levels and are dynamically controlled by bone morphogenetic proteins (BMPs)11 and factors regulating intramedullary osteoblastic cells and their progenitors.12 Early stem cells can be identified and isolated using a unique array of surface antigen-receptor expressions (CD34+/−, Thy1,lo KIT+, CD38−, CD33−, vascular endothelial [VE]-cadherin+, KDR/FLK1+, FLK2−/FLT3−, CD133+/−)13,14,15,16,17,18 and have a unique molecular signature.19,20 The ability to efflux specific chemical dyes has also been used to provide enriched populations of HSC.21,22,23,24 Isolated cell populations enriched in HSC can be quantified using in vitro long-term progenitor assays and surrogate in vivo repopulating assays in severely immunodeficient mice and xenogeneic animal models (Chap. 18).
SITES OF HEMATOPOIESIS
As shown in Fig. 5–1, the marrow is the last in a series of anatomical sites of hematopoiesis that change several times during embryonic and fetal development.25,26,27,28 The earliest hematopoietic cells develop in the blood islands of the extraembryonic yolk sac during late gastrulation and form the primitive hematopoietic system. This primitive hematopoiesis is transient, lasting from the appearance of the blood islands at embryonic days 7.5 days postcoitum (dpc) in mice and 19 dpc in humans through the final cellular divisions in the circulating embryonic blood at 13.5 dpc in mice and week 6 in humans.28,29 The large majority of primitive blood cells produced are erythrocytes that enucleate after release into the circulation, and their hemoglobin contains the embryonic α- and β-globin chains. Primitive hematopoietic cells also give rise to macrophages and megakaryocytes. Overlapping with this transient primitive hematopoiesis is definitive hematopoiesis that gives rise to all of the blood cell types found in the adult (Chap. 7).
Figure 5–1.
Expansion and recession of hematopoietic activity in extramedullary and medullary sites. For details regarding the nature of yolk sac and hepatic hematopoiesis, see “Sites of Hematopoiesis: Embryogenesis and Early Stem Cell Development.” Chapter 7 provides a more comprehensive treatment of this topic (see Fig. 7–1 in Chap. 7).
Transplantation experiments in hematopoietically ablated mice have demonstrated that definitive hematopoietic cells arise on 8.5 to 11.5 dpc in mice and weeks 4 to 6 in humans in three different embryonic locations: the yolk sac blood islands, the anterior portion of the aorta-gonad-mesonephros (AGM) region, and the allantoic portion of the developing placenta.26,27,28 The definitive murine erythroid cells circulating on 8.5 to 11.5 dpc appear to be descendent from a transient population of erythroid/myeloid progenitors derived from the yolk sac, rather than being derived from HSCs that arise in the placenta and AGM as occurs at later times in the fetus and adult.30 Serial transplantation in irradiated mice demonstrated that the earliest appearance of the intraembryonic human HSCs is in the AGM at week 5.31 HSCs migrate through the blood to the fetal liver where they seed and mature into all of the cellular elements of the blood.25,26,27,28 Erythrocytes, the predominant cell produced by definitive hematopoiesis during prenatal development, are smaller than the primitive erythrocytes, and their hemoglobin contains the fetal and adult globin chains. In mid-gestation, the HSCs that have migrated to the fetal liver undergo an exponential expansion and display a specific integrin (Mac-1) that is not found in marrow HSCs.32 In the last third of gestation, the HSCs and early hematopoietic progenitor cells migrate from the fetal liver through the circulation seeding the spleen and marrow. Fetal liver hematopoiesis declines steadily as the spleen and marrow become the major hematopoietic sites. At birth, the marrow is the major hematopoietic site in humans, while the spleen remains a prominent but decreasing site in the mouse (Chap. 7).
Visceral endoderm is in close proximity to the mesoderm formed by gastrulation in those sites where HSCs are generated in the embryo. This proximity is important in that the endoderm appears to induce both endothelial and blood cell development in the adjacent mesoderm through secretion of Indian hedgehog (IHH), a member of the hedgehog family of proteins.33 IHH, in turn, upregulates the expression of BMP4 in the developing mesodermal cells.33 BMP4 upregulation is important for the development of both the endothelial cells that form blood vessels and the HSCs located within these vessels.33,34 Developing endothelial cells and hematopoietic cells in the vessels formed by these endothelial cells are found in each site of primitive and definitive hematopoiesis. The close association of these two cell types in the developing embryo has led to the proposal for their having a common precursor, the hemangioblast.35,36 Important proteins involved in the development of the hemangioblasts are BMP4, VE growth factor receptor KDR/Flk-1, transcription factor TAL1, and TAL1’s binding partner LMO2.35,36
Marked endothelial cells in mice give rise to the HSCs.37 Imaging studies in zebrafish38,39 and mice40 indicate that specialized hemogenic endothelial cells in the ventral part of the aorta can transform without mitosis into HSCs. The differentiation of HSCs from hemangioblasts and/or hemogenic endothelium requires the signaling protein Notch1 and the transcription factors GATA-2, MYB, and Runx1.35,36,41,42 The mechanism driving this earliest expansion of HSC is not well-defined, but two factors that also play roles later, KIT ligand/stem cell factor (SCF) and interleukin (IL)-3, are important in the embryo. BMP4, in addition to its role in the induction of hematopoietic and endothelial differentiation, increases proliferative and self-renewal of HSCs33,34 as it differentially upregulates KIT (SCF receptor) in the HSCs, but not in adjacent endothelial cells.43 Expansion of the earliest definitive HSC is also mediated by Notch signaling as it induces the Runx1 transcription factor41,42 and one of its targets, the IL-3 gene.44
Primitive stem cells from human fetal liver or marrow reconstitute all lymphohematopoietic-derived cells and part of the stromal microenvironment in in vivo repopulation assays.45 These observations are consistent with the early derivation of hematopoietic, vascular, and stromal cells from a CD34−, KDR/Flk-1+, multipotential mesenchymal stem cell.14,15,16,46 Identification of AC133+, CD34−, CD7− HSCs47 and demonstration of endothelial precursors in AC133+ progenitor cells48 underscore the crosstalk between hematopoiesis and angiogenesis signaling pathways and establish the functional role of hemangioblasts in ontogeny.49,50,51 As early fetal hematopoiesis is established, the yolk sac vascular networks remain active sites of progenitor production and hematopoiesis.28 Long-term reconstituting HSCs express two members of the ATP-binding cassette genes (ABCG-2 and P-glycoprotein), allowing the efflux of mitochondrial vital dyes such as Hoechst 33342 and rhodamine 123 and their isolation by multiparameter flow cytometry based on their low side scatter (side population [SP] cells).21,22,23,24 Enrichment of the SP population for HSC has been achieved in both adult marrow52 and fetal liver53 populations by using the signaling lymphocyte and activation markers (SLAMs) to select cells with the specific phenotype (CD150+, CD244−, CD48−). Nearly half of the individual cells in the CD150+, CD244−, CD48− population provide long-term hematopoietic reconstitution in irradiated mice.52
Derivation of hematopoietic cells from adult tissue (muscle, liver) is attributed to resident marrow-derived stem cells in these tissues.54,55 A role for adult marrow-derived mesenchymal stem cells in the repair and regeneration of nonmarrow organs has been described, including cardiac and smooth muscle, liver, and brain.56,57 However, these marrow-derived mesenchymal stem cells function mainly by providing a microenvironment through various cytokines that induce cell growth and stimulate vascularization or by fusing with local cells, rather than by transdifferentiation into specific differentiated cells of the organ undergoing repair (Chap. 18).56,57
The formation of the marrow in the third trimester of mammalian prenatal development involves the circulation and chemotaxis of HSCs, which have greatly expanded their numbers in the fetal liver, to the newly developed marrow niche (see “Marrow Structure” below). The release of HSC from the murine fetal liver coincides with the progressive loss of two adhesion proteins, CD144 (VE-cadherin) and CD41 (integrin α2b).58,59 In mice, the seeding of the marrow with HSCs is first detected at 17.5 dpc,60 but the formation of the marrow niches for the HSCs and their progeny occurs in the preceding 3 days in sites of endochondral bone formation.61 Differentiation of a clonal skeletal progenitor stem cell results in cell populations that form cartilage, bone, or marrow niches that either support HSCs or the differentiating progeny of HSCs.62 The specific cells supported by a niche depend upon the expressions of endoglin, Thy1, and aminopeptidase A by the mesenchymal descendants of the skeletal progenitor stem cell. The migration of the circulating HSCs to their supporting marrow niches, which are formed by cells expressing aminopeptidase A but not endoglin or Thy1,62 is directed by the synergistic action of the chemokines CXCL12 and SCF for which the HSCs display the respective receptors, CXCR4 and KIT.60 Other chemotactic factors and adhesion molecules contribute to HSC migration from the fetal liver to the developing bone where their seeding and differentiation initiates marrow hematopoietic function in mammals.58,59,60
Cavities within bone occur in the human being at about the fifth fetal month and soon become the exclusive site for granulocytic and megakaryocytic proliferation. Erythropoietic activity at the time is confined to the liver. The microenvironment in the marrow becomes supportive of erythroblasts only toward the end of the last trimester (see Fig. 5–1). The formation of the marrow cavities in the developing mouse bones appear at a relatively later time in the prenatal life of mice than humans, and it involves an IHH-regulated63 synchronized maturation of osteoblast progenitors arising from mesenchymal stem cells and osteoclast progenitors arising from HSCs in the areas of mineralized cartilage of the fetal bones.64 Most of the marrow spaces form in the endochondral bones but some marrow develops in the intramembranous bones of the cranium and scapulae. As these respective progenitors differentiate in situ they acquire the phenotype of osteoblasts with expression of osteopontin, osteonectin, bone sialoprotein, and macrophage colony-stimulating factor (M-CSF), and of osteoclasts with expression of tartrate-resistant acid phosphatase (TRAP), calcitonin receptors, and c-FMS (M-CSF receptor).64 In the human, marrow hematopoiesis begins at the 11th week of gestation in specialized mesodermal structures termed primary logettes.65 The logettes are composed of mesenchymal cells and fibers that surround a central artery and protrude into the venous sinuses of the developing marrow cavities. The myeloid and erythroid hematopoietic cells that populate the logettes are derived not from HSCs but rather from later-committed progenitors.65 Just after birth the HSCs are found in the marrow, and hematopoiesis is evident throughout the marrow cavity.
By the fourth year of life, a significant number of fat cells have appeared in the diaphysis of the human long bones.66 These cells slowly replace hematopoietic elements and expand centripetally until, at approximately 18 years of age, hematopoietic marrow is found only in the vertebrae, ribs, skull, pelvis, and proximal epiphyses of the femora and humeri. Direct measurements of the volume of bone cavities reveal increases from 1.4 percent of body weight at birth to 4.8 percent in the adult,66 whereas blood volume decreases from 8 percent of body weight in the newborn to approximately 7 percent in the adult.67 Expansion of marrow space continues throughout life, resulting in a further gradual increase in the amount of fatty tissue in all bone cavities, especially in the long bones.68,69 Although the quantity of adipose tissue in the head and trochanteric parts of the femur varies in individuals, the fat content of this area of hematopoietic marrow progressively increases as adult humans age.70 The preference of hematopoietic tissue for centrally located bones has been ascribed to higher central tissue temperature with greater vascularity.71 In mice, an increased prevalence of adipose tissue in tail vertebrae as opposed to the more central thoracic vertebrae is associated with fewer HSCs and hematopoietic progenitors.72 Genetic absence of adipose tissue or chemical inhibition of adipocyte generation was associated with improved posttransplant hematopoietic regeneration, suggesting that marrow adipocytes are negative regulators in the hematopoietic microenvironment.72
MARROW STRUCTURE
The blood supply to the marrow comes from two major sources. The nutrient artery, the principal source, penetrates the cortex through the nutrient canal. In the marrow cavity, the nutrient artery bifurcates into ascending and descending central or medullary arteries from which radial branches travel to the inner face of the cortex. After repenetrating the endosteum, the radial vessels diminish in caliber to structures of capillary size that course within the canalicular system of the cortex. In the canalicular system, arterial blood from the nutrient artery mixes with blood that enters the cortical capillary system from the periosteal capillaries derived from muscular arteries.73 After reentering the marrow cavity, the cortical capillaries form a sinusoidal network (Fig. 5–2). Hematopoietic cells are located in the intersinusoidal tissue spaces. Some arteries have specialized, thin-walled segments that arise abruptly as continuations of arteries with walls of normal thickness.74 These vessels give off nearly perpendicular branches analogous to the arterial branching observed in the spleen and kidney, permitting volume compensation for changes in intramedullary pressure. In the marrow cavity, blood flows through a highly branching network of medullary sinuses. These sinuses collect into a large central sinus from which the blood enters the systemic venous circulation through emissary veins. Histomorphic studies of normal murine marrow demonstrate that all hematopoietic cells are within 18 μm or less than 3 cell diameters of a blood vessel.75
Vascular networks consisting of cells expressing CD31, CD34, and CD105 (endoglin) but lacking intercellular adhesion molecule (ICAM)-1, ICAM-2, ICAM-3, or endothelial leukocyte adhesion molecule (ELAM)-1 (E-selectin) can form within the stroma of long-term marrow cultures. These findings underscore the intimate relationship of blood vessels to hematopoietic activity.76 A study of early hematopoiesis of human marrow from long bones (ages 6–28 weeks) has shown an absence of CD34+ hematopoietic progenitors before onset of hematopoiesis, a predominance of CD68+ cells mediating chondrolysis, and CD34+ endothelial cells developing into specific vascular structures organized by endothelial cells and myoid cells.77 Vascular endothelial growth factor (VEGF) receptors found on CD34+ cells16 and AGM primitive stem cells underscore the common ontogeny.78 Subsets of CD34+ cells expressing the AC133 antigen and the human VEGF receptor-2 (KDR/FLK1) define the functional endothelial precursor phenotype.79 Endothelial progenitors residing in the CD34+, CD11b+ subsets are capable of producing and binding angiopoietins,80 and fibronectin (FN) enhances VEGF-induced CD34 cell differentiation into endothelial cells.81 Growth and remodeling of bone, marrow space, and the vasculature that supplies them with nutrients and oxygen are closely linked by the relative hypoxia of the marrow and surrounding bone.82 The transcription factors, hypoxia-inducible factor (HIF)-1α and -2α, are stabilized by hypoxia and increase VEGF expression in osteoblasts, and lead to regulated, coupled growth by endothelial cells and osteoblasts, both of which have VEGF receptors.82 The expansion of erythropoiesis in response to erythropoietin (EPO) in mice is associated with a reciprocal decrease in the vasculature.83
Myelinated and nonmyelinated nerve fibers are present in periarterial sheaths in the marrow,84 where they are believed to regulate arterial vessel tone. Nerve terminals are distributed between layers of periarterial adventitial cells or localize next to arterial smooth muscle cells.85 Nonmyelinated fibers terminate in the hematopoietic spaces, implying that neurohumors elaborated from free-nerve terminals affect hematopoiesis. Intimate cell–cell communication between sympathetic nerve cells and structural elements within the marrow sinuses occurs at less than 5 percent of nerve terminals that terminate within the hematopoietic parenchyma or on sinus walls. This anatomical unit, termed a neuroreticular complex, consists of efferent (autonomic) nerves and marrow stromal cells connected by gap junctions.85 The marrow is highly innervated along the arterioles and less frequently along capillaries, where neurologic control of blood flow and angiogenesis appear to be mediated via neurokinin A and substance P.86
In mammals, hematopoiesis occurs in the extravascular spaces between marrow sinuses. The marrow sinus wall is composed of a luminal layer of endothelial cells and an abluminal coat of adventitial reticular cells, which forms an incomplete outer lining (Fig. 5–3). A thin, interrupted basement lamina is present between the cell layers. Circulating HSCs move across the sinus endothelium into the extravascular space where they proliferate and differentiate into mature cells, which move across the sinus endothelium and circulate in the blood. Nonhematopoietic cells and extracellular matrix in the extravascular space form the marrow stroma. Stromal cells obtained from animal or human marrow can be studied in cultures,87 are derived from fibroblasts, and have unique phenotypic and functional characteristics that allow them to nurture hematopoietic development in highly specialized microenvironmental niches.88 However, newer studies with mutant mice and mice with specific cells that can be identified by direct fluorescence microscopy89 have led to an understanding of the spatial orientation of the stroma and the localization of hematopoietic niches that they form in the marrow.
Figure 5–3.
Transmission electron micrograph of a mouse marrow sinus. The small arrow in the sinus lumen (L) indicates the perikaryon of an endothelial cell. Several endothelial cell junctions are present along the circumference of the sinus endothelial wall. Thus, the wall is composed of the cytoplasm of endothelial cells that overlap or interdigitate. Two adventitial reticular cell bodies are identified by arrows at the top and upper left of the sinus. The cytoplasm of the adventitial reticular cells is discontinuous as it is followed around the sinus. Three cytoplasmic processes of adventitial reticular cells are indicated by arrows. Other, smaller processes of reticular cell cytoplasm are found upon close inspection of the sinus periphery and the hematopoietic spaces. The scattered rough endoplasmic reticulum and dense bodies are characteristic of the reticular cell cytoplasm. (Reproduced with permission from Lichtman MA: The ultrastructure of the hemopoietic environment of the marrow: A review. Exp Hematol. 9:391, 1981.)
The hematopoietic niche concept was originally described for an operationally defined murine multipotential pluripotential progenitor (MPP) in the spleen,90 but it has been extended to various marrow hematopoietic subpopulations, including physically demonstrated niches of HSCs,91 lymphoid cells,92,93 and erythroid cells.93,94 The cellular components of these hematopoietic areas of the marrow include several types of nonhematopoietic cells including: (1) the sinus endothelial cells, (2) mesenchymal stem cells (MSCs) that form the skeletal elements of bone and marrow space such as chondrocytes, osteoblasts, osteocytes, fibroblasts, and adipocytes, and (3) terminally differentiated cells of hematopoietic origin such as macrophages, lymphocytes, and plasma cells. Experiments in both mice61 and humans95 have demonstrated by heterotopic bone formation that host marrow sinusoidal endothelial cells and hematopoietic cells will infiltrate and develop within microenvironment provided by a transplanted MSCs and its progeny. In mice, these MSCs are identified by a CD105+, Thy1−, 6C3− phenotype, which can support specific hematopoietic populations as their progeny develop Thy1 and 6C3 expression.62 In humans, these MSCs are identified as CD45−, CD146+ adventitial reticular cells with fibroblast colony forming capacity that can interconvert between this MSC status and CD146− chondrocytes.96
Studies localizing marrow areas that support murine HSCs and their early progeny the hematopoietic progenitor cells (HPCs) have led to the concept of two niches for these hematopoietic cells: an endosteal niche that promotes HSC quiescence and a vascular/perivascular niche that is associated with self-replicating HSCs.97 Studies combining vascular and endosteal imaging demonstrate that HSC/HPCs localized in the endosteal areas were also within a few cell diameters of VE cells.75,98 The hypoxic status of HSC/HPCs, in terms of HIF expression, is unrelated to their proximity to blood vessels,98 the flow rate of blood in the marrow vessels in the vicinity of HSCs appears to be very low,99 and the lowest oxygen tension directly measured in the marrow is in the perivascular areas of microvessels.100 These results suggest that the functional status of microvessels has a larger role in HSC niche activity than the proximity of the potential niche to its vascular supply.
Endothelial cells are broad flat cells that completely cover the inner surface of the sinus.101 They form a major barrier and control the system for chemicals and particles entering and leaving the hematopoietic spaces, with overlapping or interdigitating unions permitting volume expansion.102 The endothelium of marrow sinusoids is actively endocytic and contains clathrin-coated pits, clathrin-coated vesicles, lysosomes, phagosomes, transfer tubules, and diaphragmed fenestrae.103,104 Marrow endothelial cells express von Willebrand factor protein,105 type IV collagen, and laminin.106 They also constitutively express adhesion molecules: ICAM-3,107 vascular cell adhesion molecule (VCAM)-1, and E-selectin,108 all of which regulate HSC proliferation.109 The distribution of sialic acid and other carbohydrates on the luminal surface of marrow sinus endothelium is discontinued at diaphragmed fenestrae and coated pits, suggesting such glycosylation plays a role in endothelial membrane function and cellular interactions.110 In vivo, the conditional deletion in endothelial cells of gp130, the common receptor component for several cytokines, including IL-6, leads to a hypocellular marrow as mice age.111 The loss of gp130 from marrow endothelial cells affects the progenitor cell populations rather than the HSC leading to a lethal anemia, a leukocytosis, but normal platelets.108
Marrow endothelial cells via direct cell–cell contacts and secreted peptides uniquely influence osteoprogenitor cell differentiation112 and regulate hematopoiesis.113 Marrow microvascular endothelium has major roles in osteogenesis through its physiologic production of the VEGF164 isoform as well as multiple cytokines that are usually associated with inflammation.114 Other marrow endothelial cell cytokines that affect hematopoiesis include SCF,115 angiopoietin-like protein 3,116 IL-5,117 thymosin β4, AcSDKP,118 and B-type natriuretic peptide.119 Endothelial cells also regulate cellular trafficking into and out of the marrow sinusoidal spaces by altering their permeability and reorganizing their cytoskeleton by ICAM-3, by VE-cadherin–mediated cell–cell contacts,107,120 and via specialized heparin sulfate proteoglycans,121 CXCL12 bound to surface proteoglycans,122 and other chemokines/chemokine receptors,123,124 such as fractalkine, a membrane-bound chemokine with a mucin stalk expressed in activated vascular beds.125 Marrow sinusoidal endothelium specifically expresses hyaluron and sialylated CD22 ligands, which are homing receptors for recirculating HSCs75 and B lymphocytes,126 respectively.
The abluminal or adventitial surface of the vascular sinus is composed of reticular cells.101,127,128 The reticular cell bodies are contiguous with the sinus, forming part of its adventitial coat (see Fig. 5–3). Their extensive branching cytoplasmic processes envelop the outer wall of the sinus to form an adventitial sheath. The sheath is interrupted and is estimated to cover approximately two-thirds of the abluminal surface area of sinuses. The reticular cells synthesize reticular (argentophilic) fibers that, with their cytoplasmic processes, extend into the hematopoietic compartments and form a meshwork on which hematopoietic cells rest (Figs. 5–4 and 5–5). The cell bodies, their broad processes, and their fibers constitute the reticulum of the marrow.
Figure 5–4.
Scanning electron micrograph of rat marrow sinus. The floor of the lumen (L) is indicated. The arrow on the left indicates the cell body of an adventitial reticular cell, which is just beneath the endothelial cell layer. Reticular cell processes can be seen coursing between the sinus wall and the hematopoietic compartment (small arrows). (Reproduced with permission from Lichtman MA: The ultrastructure of the hemopoietic environment of the marrow: A review. Exp Hematol. 9:391, 1981.)
Figure 5–5.
Scanning electron micrograph of rat femoral marrow sinus. The lumen (L) of an exposed sinus that has been cut open is indicated. The single asterisk indicates the process of an adventitial reticular cell and the intimate contact it makes with a hematopoietic cell. To the left of this process are adventitial reticular cell fibers, which form a scaffold for hematopoietic cells. The double asterisk identifies a portion of a reticular cell. The hole in the sinus floor is an artifact of preparation or a migration channel bereft of the emigrating cell. Empty spaces between cells and fibers are artifacts of preparation. The arrow to the left points to thin-walled fenestrae in the endothelial cytoplasm. The arrow to the right identifies the portion of a reticulocyte that may be penetrating the sinus wall, early in egress (see Fig. 5–8A). (Used Lichtman MA, University of Rochester.)
Adventitial reticular cells can differentiate along the smooth muscle pathway and contain α smooth-muscle actin, vimentin, laminin, FN, and collagens I, III, and IV.129,130 More specialized contractile reticular “barrier cells” have been described in mouse marrow after hematopoietic stress.131 Barrier cells increase in number and seem to enclose small vessels and extend the venous sinuses so that release of precursors is restrained while accommodating an increased entry of mature cells into the circulation.131
Studies in both mice and humans have identified subsets of adventitial reticular cells as MSCs with adipocytic-osteogenic potential that in mice appear to have significant overlap: (1) CXCL12–abundant reticular (CAR) cells,132 (2) adventitial reticular cells expressing the intermediate filament protein Nestin and displaying the surface proteins platelet-derived growth factor (PDGF) receptor-α and CD51 (Nestin+, PDGFRα+, CD51+),133 and (3) adventitial reticular cells expressing leptin receptors.115,134 The human equivalents are a population of CD45−, CD146+ adventitial reticular cells that have smaller subsets that express Nestin, PDGFRα, and CD51.133 A major proportion of these subsets are restricted to the perivascular area, but have some cells scattered throughout the hematopoietic marrow. However, because these adventitial reticular subsets are also the major sources of CXCL12 and SCF in the marrow, they have important roles in establishing the HSC niche near the marrow microvasculature while their progeny establish the endosteum and its associated hematopoietic niche in the marrow.
The majority of CAR cells are in close association with the sinusoidal endothelial cells but some are also associated with the endosteum. Like the Nestin+ MSCs, CAR cells appear to be progenitors of osteoblasts and adipocytes while producing major amounts of CXCL12 and SCF.135 Development of CAR cells and their production of CXCL12 and SCF is associated with the expression of the transcription factor Fox1c.136 CAR cells and the niches that they create in the marrow are required for the normal development of HSC, various differentiation stages of B-lymphocytes, natural killer cells, and the plasmacytoid dendritic cells that are all found in close physical association with CAR cells.137
Autonomic neurons innervate the perivascular Nestin+, PDGFRα+, CD51+ adventitial reticular cells which maintain the HSC niche by several surface-displayed and/or secreted products including IL-7 and VCAM-1, in addition to SCF and CXCL12.138 β-Adrenergic neurotransmission inhibits the expression of these proteins so that mice with specific denervation have decreased marrow cellularity and increased circulating hematopoietic progenitors.139 The sympathetic nervous system controls circadian fluctuations in circulating HSC numbers though its effect on MSC expression of the chemokine CXCL12 in the marrow.140 Studies in mice with defective myelinization and mice treated with adrenergic antagonists or agonists indicate that the adrenergic nervous system in the marrow also regulates mobilization of HSCs by granulocyte colony-stimulating factor (G-CSF).141 However, the nonmyelinating Schwann cells associated with the autonomic nerves of the marrow secrete transforming growth factor-β (TGF-β) and thereby maintain the HSC quiescence.142
Adipocytes in the marrow develop by lipogenesis in fibroblast-like cells (Fig. 5–6). Reticular cells in mouse and human marrow can undergo transformation to fat cells in vitro and can revert into fibroblasts in culture by lipolysis,101,143 and the Nestin+ MSCs138 and CAR MSCs can differentiate into adipocytes. A reciprocal relationship between adipocyte and osteoblast differentiation of MSCs appears to be controlled by multiple transcription factors, with both peroxisome proliferator-activated receptor-γ2 (PPARγ2)144 and CCAAT/enhancer binding protein (C/EBP)145 promoting adipocyte differentiation. Marrow fat cells are relatively resistant to lipolysis during starvation, and their phenotype is consistent with both white and brown fat.146,147 Although the proportion of saturated fatty acids is lower than in other fat deposits, marrow fat composition depends on whether it is located in the red, hematopoietically active, or the yellow, hematopoietically inactive, marrow. Human marrow adipocytes support the differentiation of late-stage, committed, myeloid and lymphoid hematopoietic cells, but they are unable to support earlier progenitor stages.148 Quantification of immature hematopoietic cells including HSCs shows reduced numbers in human marrows with increased fat,70 and in vivo studies in mice confirm that marrow adipocytes create a negative hematopoietic microenvironment that reduces development of HSCs and early-stage common hematopoietic progenitors.72
Figure 5–6.
Scanning electron micrograph of rat femoral marrow. Several sinuses and the intervening hematopoietic cords are evident. The exposed lumen (L) of one branching sinus is indicated. The sinus, just above the L, contains a bean-shaped proplatelet with an attenuated strand connected to a separating smaller proplatelet fragment. Smaller proplatelet fragments are below the L. The short horizontal arrow points to the cytoplasm of a transected megakaryocyte. The lower arrow points to a fat cell. The rat femoral marrow contains a modest number of fat cells. Spaces in the hematopoietic cords are artifacts resulting from transecting the femur. (Reproduced with permission from Lichtman MA: The ultrastructure of the hemopoietic environment of the marrow: A review. Exp Hematol. 9:391, 1981.)
Osteoblasts, osteoclasts, and elongated flat cells with a spindle-shaped nucleus form the marrow endosteal lining.149 These endosteal cells and the closely associated microvascular cells participate in a dynamic process in which endochondral bone formation proceeds with removal of calcified cartilage and connective tissues by macrophages while new bone is formed by osteoblasts and remodeled by specialized osteoclasts.114,150 Osteoblasts that become embedded in the bone matrix proteins are termed osteocytes, a terminally differentiated cell that has secretory capacity and influences the activities of osteoblasts, osteoclasts, and hematopoietic cells. Resting endosteal cells express vimentin, tenascin, α smooth-muscle actin, osteocalcin, CD51, and CD56. They do not react with antibodies to CD3, CD15, CD20, CD34, CD45, CD68, or CD117.151 Enriched CD56+, CD45−, CD34− endosteal cells grown in the presence of cytokines (insulin growth factor I, basic fibroblast growth factor [bFGF], SCF, IL-3, granulocyte-macrophage colony-stimulating factor [GM-CSF]) do not give rise to hematopoietic cells, which suggests they are not totipotent MSCs.151 In the next sections, the two major types of cells responsible for endosteal activity, osteoblasts and osteoclasts, are considered in terms of their potential roles in maintaining the hematopoietic niche.
Osteoblasts have three major functions: formation of new bone by regulating the secretion of the bone matrix proteins, regulation of bone resorption via osteoclast activity, and regulation of the hematopoietic environment mainly by secretion of cytokines. Bone-forming osteoblast progenitor cells, like stromal precursors, reside in the CD34−, STRO-1+ nonadherent marrow cell population.152,153 The differentiation of mesenchymal cells into either osteoblasts or adipocytes is related to the relative activities of Runx2 and PPARγ, respectively.154 With aging, the sensitivity to PPARγ appears to increase, contributing to the increase in adipose tissue in the marrow found with older age.154 BMP2,155 bFGF,156 hepatocyte growth factor (HGF),157 parathyroid hormone12 and endothelin-1158 promote osteoblast growth, whereas the cytokine TGF-β159 and the transcription factor osterix160 promote differentiation. Osteoblasts increase early hematopoietic progenitor survival in long-term cultures and secrete hematopoietic growth factors such as M-CSF, G-CSF, GM-CSF, IL-1, and IL-6.161,162 Osteoblasts also produce various cytokines such as hematopoietic cell-cycle inhibitory factors TGF-β,163 osteopontin,164 and CXCL12,12 as well as cell-cycle stimulatory factor Dickkopf-1,165 all of which contribute to stem cell regulation within the marrow microenvironment. Direct cell–cell communication has been shown in the marrow and in osteoblastic cell networks,166 indicating a potential regulatory role for anatomical gap junctions in hematopoiesis.167,168 The size of stem cell niches increases after osteoblastic expansion and Notch activation in transgenic models.11,12 In another model, intramedullary hematopoiesis and stem cell numbers are severely diminished following in vivo ablation of osteoblasts,169 underscoring the importance of this cell type to the marrow hematopoietic inductive microenvironment. The lymphoid niches for early lymphoid progenitors and differentiating B cells are located adjacent to the endosteal surface.92,93 Osteocytes, which are terminally differentiated osteoblasts trapped in the bony matrix, secrete cytokines into the marrow space that act in a negative feedback manner on new bone formation. Specifically, the osteoblast and stromal cell surface protein receptor activator of nuclear factor-κB ligand (RANKL) activates osteoclasts,170 while the cytokine sclerostin suppresses osteoblast activity.171 Disruption of the signaling mechanism of G-protein receptors in osteocytes leads to an expansion of myelopoiesis that is mediated by secreted myelopoietic cytokines, most likely G-CSF.172
Mature osteoclasts are multinucleated giant cells derived from fusion of progenitor cells of the monocyte/macrophage lineage of the HSC.173 The mature osteoclasts resorb and remodel bone, regulate osteoblast activity, and help control the HSC entry into and exit from the marrow.174,175 The osteoclasts have motile and resorptive phases. They require the Wiskott-Aldrich syndrome protein during clustering and fusion of actin-based adhesion structures named podosomes.176 Podosomes are involved in the formation of specific structures termed sealing zones in which actin rings surround an area of ruffled plasma membrane at the face of the endosteal bone. Within these sealing zones, osteoclasts secrete hydrochloric acid and digestive enzymes that resorb bone. Osteoclasts also can be derived from pro-B cells, as shown by Pax-5 knockout mice, which have increased osteoclasts and severe osteopenia.177 When osteoclast activity or number are reduced or eliminated in mice through null mutations or homologous recombination, the marrow cavities fail to form resulting in osteopetrosis. Based on studies of osteopetrotic mice, proteins required for osteoclast differentiation include the macrophage transcription factor PU.1; the secreted and surface displayed cytokine M-CSF of stromal cells and its receptor c-FMS on osteoclasts; the transcription factor c-FOS; the cytokine RANKL; its osteoclast receptor RANK, the signaling transducer tumor necrosis factor-α (TNF-α) receptor-associated factor 6 (TRAF 6); the downstream transcription factor nuclear factor (NF)-κB, and nuclear factor of activated T cells (NFAT).175,178,179 Other osteopetrotic mice strains have deficiency of proteins required for the bone resorption function of osteoclasts. These proteins include the β3 component of the αvβ3 integrin (vitronectin receptor) required for binding of the osteoclast sealing zone to bone; c-Src signaling protein; the proton transporting H+ adenosine triphosphatase (ATPase) and chloride channel protein required for HCl secretion; and the secreted osteoclast proteins cathepsin K, matrix metalloproteinases, and TRAP that digest the bone matrix.174,175,179
Osteoblast/stromal cells regulate differentiation of osteoclasts through intimate cell–cell contacts. They are found in direct apposition to osteoclasts with coated pit formation, suggesting accumulation of receptor–ligand complexes in endocytic vesicles.180,181 The recruitment of the osteoblasts and osteoclasts appears to be through capillaries associated with the remodeling compartment.182 A major regulatory mechanism by which osteoblasts and osteoclasts interact is the RANK/RANKL/osteoprotegerin (OPG) system of signaling.182 Osteoclast differentiation and maturation require the signaling cascade from RANK on the cell surface through TRAF 6, NF-κB, and NFAT.180 Osteoblasts and their progenitor cells display RANKL on their surfaces, and binding of RANKL to the RANK on the osteoclasts and their progenitors promotes differentiation and activation of the osteoclasts. Osteoblasts also secrete OPG, a decoy receptor for RANKL, which inactivates RANKL by binding to the active site of RANKL, thereby preventing its binding to RANK. As a result, osteoclastic activity is decreased when OPG concentrations are high and increased when they are low.183 Another signaling mechanism by which osteoclasts and osteoblasts reciprocally regulate the differentiation and activities of each other is the ephrinB2-EphB4 signaling system.184 Osteoclasts express ephrinB2 on their surfaces while the osteoblasts express EphB4, a member of the receptor tyrosine kinase (RTK) family, which is the receptor for ephrinB2. Binding of ephrinB2-EphB4 results in bidirectional signaling in which osteoclast differentiation is decreased though suppression of the c-FOS–NFATc1 activity, whereas osteoblast differentiation is increased by EphB4 signaling.184
Osteoclasts produce HGF and express c-Met, the HGF receptor, implying a paracrine and autocrine regulatory pathway between them and adjoining osteoblasts.157,185 Similarly, blocking expression of cadherin-6 interferes with heterotypic interactions between osteoclasts and stromal cells, impairing their ability to support osteoclast formation.186 CD9, a tetraspanin transmembrane adhesion protein on stromal cells,187 influences myelopoiesis in long-term marrow cultures.188 Inhibition of stromal cell CD9-mediated signaling by a blocking antibody reduces osteoclast differentiation factor transcription, leading to reduced osteoclastogenesis.189 Macrophage-stimulating protein, a HGF-like protein, signals through the stem cell-derived tyrosine kinase, a member of the HGF receptor family. It also stimulates osteoclast bone-resorbing activity by enhanced cytoskeletal reorganization without affecting proliferation of osteoclast precursors.190,191 Osteoclast differentiation is influenced by monocytes expressing ADAM-8 (CD156), a protein of the disintegrin and metalloproteinase family,192 and eosinophil chemotactic factor-L (ECFL),193 characterizing complex cell–cell, cell adhesion protein, stromal cell cytokine, and chemokine signals within the marrow microenvironment.
Lymphocytes, including T, natural killer (NK), B, and plasma cells, and macrophages, including monocyte-derived, antigen-presenting dendritic cells, arise from the HSCs and undergo part of their differentiation in the marrow. They then circulate and, in the case of the lymphoid cells, reside and further differentiate in other organs such as the thymus, spleen or lymph nodes, before returning to the marrow, where they terminally differentiate and form part of the marrow microenvironment by producing growth factors (IL-3, CCL3) and participating in cell–cell interactions with developing progenitors.84,101,194 Monocytic/macrophage progenitor cells can enter the circulation and later enter many different tissues where they differentiate into macrophages. In the marrow, the monocytic/macrophage progenitors can differentiate into macrophages or fuse and become osteoclasts. Lymphocytes and macrophages concentrate around arterial vessels, near the center of the hematopoietic cords. B cells also cluster near the osteal surface.92,93 Mature B and T lymphocytes in the marrow are in contact with a specific set of monocyte-derived, antigen-presenting dendritic cells that are clustered around the blood vessels.195
Lymphocytic differentiation begins as HSCs that have committed to differentiation as multipotent HPCs (MPPs) lose their potential to become megakaryocytic-erythroid progenitors (MEPs) and granulocyte-macrophage (GM) progenitors; this change in differentiation potential is detectable as the upregulation of lymphoid-specific transcripts, that is, they are lymphoid-primed multipotent progenitors (LMPPs). The commitment of LMPPs to lymphoid differentiation in these early-stage HPCs is reinforced by progressive expression of FMS-like tyrosine kinase 3 (Flt-3), IL-7 receptors (IL-7R), and recombination activating genes-1/2 (Rag-1/2) proteins.196,197 These early lymphoid progenitors (ELPs) require a microenvironment provided by MSCs and their osteogenic progeny which supplies VCAM-1, CXCL12, Flt-3 ligand, and IL-7.198,199 The ELPs enter the blood with transit to the thymus where they undergo differentiation into T cells. In addition, to its role as site of early T-lymphocyte development, the marrow acts a secondary organ for the proliferation of mature CD8 and CD4 memory T lymphocytes. Although no specific organized structure or niche has been found for these T lymphocytes, they can represent up to 4 percent of nucleated cells in the marrow that they reenter by migrating through the sinusoidal endothelium from the blood.200 Alternatively, LMPPs can remain in the marrow and differentiate into common lymphocyte progenitors (CLPs) that give rise to NK progenitor cells, which differentiate in the marrow, or prepro-B cells that mature to the pro-B cells, which migrate from the marrow to the lymph nodes or spleen where they differentiate further.196,197
Marrow stromal cells facilitate the maturation of NK cells,201 an effect likely mediated by stromal-derived Flt-3 ligand and IL-15.202 Within the marrow, both NK cells and CD8+ memory T cells require the coordinated expression of secreted IL-15 and surface IL-15 receptors by other marrow cells for their survival and development.203 The marrow MSCs and their osteogenic progeny also create a microenvironment for proliferation and differentiation of ELPs through the later sequential lymphoid stages of CLPs, prepro-B cells, pro-B cells, and pre-B cells via the provision of osterix and galectin-1.198
The differentiation stages subsequent to the pro-B cells occur after the cells enter the blood and seed the lymphoid follicles of the secondary lymphoid organs, mainly spleen and lymph nodes. From these lymphoid organs, the cells then reenter the blood as B lymphocytes or immature plasma cells. The immature plasma cells that have differentiated in the spleen and will become the long-lived plasma cells return home to the marrow, where they are located in contact with CXCL12-producing stromal cells. A negative feedback is completed as the mature plasma cells either compete with the prepro-B cells for sites on the CXCL12-producing stromal cells or directly induce apoptosis of the prepro-B cells.204 Marrow blood vessel-associated dendritic cells produce macrophage migration-inhibition factor, a cytokine required for survival of mature B lymphocytes that have matured in secondary lymphoid organs and recirculated to the marrow.195
Hematopoietic progenitors restricted to monocyte/macrophage differentiation are characterized by expression of M-CSF receptors (FMS), membrane-activating complex-1 (CD11b), and F4/80 antigen, and give rise to monocytes that enter the blood.205 These nondividing monocytes can then enter various organs, including a subset with high Ly6C that reenter the marrow where they become macrophages and antigen-presenting dendritic cells.205,206 Although they are both descendants of similar M-CSF–dependent monocytic progenitors, macrophages differ from osteoclasts by their single nucleus and, in mice, expression of F4/80 antigen as well as lack of TRAP and calcitonin receptors.206 Marrow macrophage phenotype207 is regulated by adjoining stromal cell–accessory cell–derived colony-stimulating factors and cytokines,208 such as M-CSF–induced upregulation of α4β1– and α5β1-integrin expression209 and Flt-3 ligand-promoting macrophage outgrowth with B-cell–associated antigens.210 Macrophages are an integral component of the local microenvironment and regulate hematopoiesis via a complex array of dual-acting stem cell stimulatory and inhibitory factors, such as IL-1, CCL3, TNF-α, and TGF-β.211,212,213 Macrophages respond to PDGF by upregulating IL-1 secretion and thereby activating primitive hematopoietic cells.214 Macrophages also modulate the structure and composition of the extracellular matrix (ECM) and its FN content.215
Specialized macrophages termed osteomacs form a canopy over the active osteoblasts and osteoclasts on the endosteal surface, where the macrophages coordinate the bone-forming activity of osteoblasts and bone-resorbing activity of osteoclasts.206 Another subset of macrophages, which are identified by CD169 (sialoadhesin/Siglec-1 [sialic acid-binding immunoglobulin-like lectin-1]), act to retain in the marrow those HSCs and early progenitor cells that are capable of circulation in the blood.216 CD169-expressing macrophages also comprise the central macrophages of erythroblastic islands that interact directly with erythroid cells,217 enhancing their proliferation and differentiation. Similarly, mature B and T lymphocytes in the marrow are supported in the specific microenvironment provided by monocyte-derived, antigen-presenting dendritic cells that are clustered around the blood vessels.195
Mesenchymal cells forming the cellular stroma in the marrow create a network of ECM proteins, such as proteoglycans or glycosaminoglycans (GAGs), FN, tenascin, collagen, laminin, and thrombospondin (TSP).218,219,220,221 Localizing signals are provided by stromal–ECM and hematopoietic cell adhesive interactions222,223 in concert with chemokines224 and cytokines bound to heparin-like structures in the GAGs.225 The binding of specific cytokines may enhance the activity of a cytokine if the GAG-binding site does not interfere with the site that binds the cytokine receptor, whereas GAG-binding sites that overlap or interfere with a cytokine receptor-binding site can inhibit the cytokine function.225 Table 5–1 lists the cytokines that are presented on the surface of stromal cells and matrix-binding chemokines and cytokines.225,226,227,228,229,230,231,232,233,234,235,236,237,238
Cell Membrane | Matrix Association |
---|---|
Chemokine | Chemokine |
Fractalkine | RANTES, PF-4, IP-10, IL-8 |
Macrophage inflammatory proteins (MIP-1α, MIP-1β) | |
CXCL12/stromal cell-derived growth factor-1 (SDF-1α, SDF-1β) | |
Monocyte chemoattractant protein-1 (MCP-1) | |
Cytokine | Cytokine |
c-KIT ligand | Granulocyte-macrophage colony-stimulating factor |
Tumor necrosis factor-α (TNF-α) | Interferon-γ (IFN-γ) |
Interleukin-1 (IL-1) | Leukemia inhibitory factor (LIF) |
Macrophage colony-stimulating factor (M-CSF) | Interleukins (IL-1α, IL-1β, IL-2, IL-3, IL-4, IL-5, IL-6, IL-7, IL-12) |
Basic fibroblast growth factor (bFGF) | |
Transforming growth factor-α (TGF-α) | Hepatocyte growth factor (HGF) |
Transforming growth factor-β (TGF-β; binding to endoglin and heparan sulfate) |
In addition to its supply of hematopoietic growth factors, the ECM provides noncellular binding partners for surface adhesion molecules of the hematopoietic and mesenchymal cells. The marrow microelasticity, which is a function of cellular density and ECM composition, varies more than 100-fold from the soft central areas to the much stiffer endosteal areas.239 This microelasticity determines the differentiation of MSCs,240 and the fate of HSCs and committed hematopoietic cells.241 In HSCs and HPCs, the activities of two nonmuscle myosin isozymes are regulated in response to the elasticity of the ECM. The increased relative activity of nonmuscle myosin II B that mediates asymmetric cell division and self-renewal is greatest in the stiffer ECM of endosteal areas, whereas increased relative activity of non-muscle myosin IIA in the areas of softer ECM mediates symmetric cell division and differentiation.239
Proteoglycans are polyanionic macromolecules (heparan sulfate, dermatan, chondroitin sulfate, hyaluronic acid) that are distributed on the surface of adventitial reticular cells and within the ECM.218,242 Heparan sulfate is the main cell-surface GAG in long-term marrow cultures, and chondroitin sulfate is the major secreted species.243 D-xylosides, which stimulate artificial sulfated GAG synthesis, increase chondroitin sulfate synthesis and hematopoietic cell production.243 Hyaluronic acid and chondroitin sulfate-containing proteoglycans are prominent in the adherent and nonadherent compartments of long-term marrow cultures.242 Heparin-containing and heparan sulfate-containing proteoglycans interact with laminin and type IV collagen244 and may play a role in cell–cell interactions, cytokine presentation, and cell differentiation.245,246,247 They also mediate progenitor cell binding to other ECM molecules such as FN.248,249,250
Agrin, a proteoglycan associated with neuromuscular junctions, is produced in the marrow by MSCs, osteoblasts and monocytes, and interacts through α-dystroglycan receptors on HSCs251 and their progeny as they differentiate along the monocyte/macrophage lineage.252 Agrin-deficient mice have hypocellular marrows as a result of decrease in all marrow hematopoietic cell lineages251 as well as specific inhibition of numbers and phagocytic function of monocytes and macrophages.252 An important hematopoietic cell proteoglycan, CD44, has hyaluronate as a major matrix ligand. The CD44–hyaluronate interaction is greatly enhanced by various cytokines, and it promotes other matrix and cellular interactions by hematopoietic cells.253 Cytokines (GM-CSF, IL-3, SCF) rapidly induce CD44 expression and increase CD44-mediated adhesion of CD34+ hematopoietic progenitors to hyaluronan.254 Lymphocyte CD44 has a binding site on the carboxy-terminal heparin-binding domain of FN,255 and neutralizing antibodies to CD44 inhibit hematopoiesis in long-term marrow cultures.256 Chondroitin sulfates A and B mediate monocyte and B-cell activation via a CD44-dependent pathway.257 Hyaluronate binding enhances hematopoiesis by releasing IL-1 in a CD44-dependent manner and IL-6 by a CD44-independent pathway.258 In humans, a specific CD44 isoform displayed on hematopoietic cells is a ligand for E- and L-selectins and plays a role in HSC homing and integrin-mediated transendothelial migration in the marrow.259
Heparan sulfate mediates IL-7–dependent lymphopoiesis235 and modulates hematopoiesis and stromal cell–matrix remodeling260 by anchoring HGF236,261 and bFGF.260,262,263 On the surface of marrow stromal cells, the main heparan sulfate-containing proteoglycans are syndecan-3, syndecan-4, and glypican-1. In the ECM, the most prevalent form is perlecan.264 Syndecan-3 is expressed by marrow stromal cells as a variant form with a core protein of 50 to 55 kDa, suggesting syndecan-3 plays a role in hematopoiesis.264 Perlecan promotes bFGF receptor binding and mitogenesis, and can bind GM-CSF.257,265 Heparan sulfate expression is induced on the cell surface in early erythroid differentiation of multipotential HSC.266 Glypican-4, another member of this family, is found on marrow stromal cells and progenitor cells.267 Syndecan-1 expression in B lymphoid cells is reduced by IL-6, which implies similar regulatory pathways in other cell types.268 Biglycan, a matrix glycoprotein, with homology to osteonectin, and the molecule SIM, a transmembrane protein, selectively increase IL-7–dependent proliferation of B cells.269 Interactions of B cells with other components of the immune system are mediated by syndecan-4, which facilitates the formation of dendritic processes270 and regulates focal adhesion, stress fiber formation, and cell migration.271
FN localizes at sites of attachment of hematopoietic cells and marrow stromal cells in vitro219,272 and at sites of interaction between these cells and developing granulocytes or monocytes.273 Early erythroid progenitors bind FN through their integrin receptors α5β1 and α4β1.274,275 Adhesion of HPCs to stroma is partly mediated by FN.248,276 The alternatively spliced form of FN (type III connecting segment [IIICS]), which is expressed uniquely within the marrow microenvironment, associates with the α4β1-integrin receptor on HSC.277 Additional IIICS FN variants have been detected in marrow stroma, providing for finely controlled progenitor–stem cell interactions based on messenger RNA splicing.278 FN adhesion to peptide domains, such as the CS1 domain (which activates α4 integrins) or stromal cells, can have dual effects of stimulation and inhibition of hematopoietic progenitor growth.279,280,281,282
The very-late antigens (VLA)-4 and VLA-5 (α4β1 and α5β1) and CD44 cooperate to promote FN adhesive interactions.279,282,283,284 Cytokines such as IL-3, SCF, and thrombopoietin (TPO) augment the magnitude of FN-mediated HPC adhesion and migration.285,286,287,288 Functional effects of FN within the marrow ECM include decreased erythroblast FN adhesion as differentiation progresses274,283 with modulation of erythroid cell differentiation dependent upon competing binding of α4β1 integrin with FN in the ECM and with central macrophages in erythroblastic islands.289 Binding of collagen I in the marrow ECM by megakaryocytes leads to their spreading and inhibition of proplatelet formation by a mechanism involving FN induction and secretion with polymerization via cosecreted factor XIII-A.290 FN is required for expression of gelatinase in macrophages291 and regulates cytokine release by M-CSF–activated macrophages292 and chondrocytes.293
The fibrillar glycoprotein tenascin-C is found in the microenvironment surrounding maturing hematopoietic cells.218,294 Tenascin-C has distinct functional domains that promote hematopoietic cell adhesion to ECM proteins or mediate a strong mitogenic signal to marrow mononuclear cells.295 Although tenascin-C–deficient mutant mice appear to have normal steady-state hematopoiesis, colony-forming capacity of marrow is markedly decreased,296 marrow regeneration capacity after cytoreductive agents is decreased,297 and retention of T-lymphocyte progenitors is impaired.297 This last effect is mediated through the α9β1 integrin on T-lymphocytes progenitors, but effects on HSCs and early hematopoietic progenitors is mediated by a different mechanism.297 Mutant tenascin-C–deficient animals also display decreased FN in their marrow, suggesting a possible mechanistic interaction between tenascin-C and FN in the marrow microenvironment.298
Collagen types I and III are associated with microvascular walls, whereas collagen type IV is confined to basal lamina beneath endothelial cells.160,299 Marrow-derived capillary networks grow in collagen gel cultures,300 inhibition of collagen synthesis reduces hematopoiesis in vitro,301 and collagen-based scaffolds are most effective for in vitro three-dimensional models of the hematopoietic microenvironment.302 Marrow-derived fibroblasts and stromal cells synthesize collagens I, III, IV, V, and VI.303 Collagen VI binds von Willebrand factor and is a strong cytoadhesive component of the marrow microenvironment.304 Erythroid and granulocytic progenitors adhere to collagen type I in vitro.305 Collagen type XIV, another fibril-associated collagen, promotes hematopoietic cell adhesion of myeloid and lymphoid cell lines.306 In situ immunolocalization of ECM proteins in murine marrow shows that collagen types I and IV and FN localize to the endosteum.307 Megakaryocyte binding to collagen I that induces FN secretion and polymerization290 enhances the α2β1-mediated collagen binding by megakaryocytes, permitting increased megakaryocyte adhesion and migration,308 which are also mediated by other megakaryocytic collagen receptors including glycoprotein VI and discoid domain receptor 1(DDR1).309
Laminins, multidomain glycoproteins with mitogenic and adhesive sites, are major components of the ECM and basement membranes.310 Laminin interactions with collagen type IV and basement membrane components such as proteoglycans and entactin311 regulate leukocyte chemotaxis.312,313 CD34+ granulocytic progenitors,314 mature monocytes,315 and neutrophils316 adhere to laminins. The role of laminins within the cytomatrix may be to strengthen adhesive interactions with α5β1 (VLA-5) and α6β1 (VLA-6) on hematopoietic cells.316 In combination with FN in vitro, laminins can expand both HSCs and several more differentiated progenitors.317 Laminins are composed of α, β, and γ polypeptides with expression of laminin-2 (α2β1γ1), laminin-8 (α4β1γ1), and laminin-10 (α5β1γ1) in the marrow.318 Stromal cells in cultures and cytokine-expanded CD34+cells also express laminin β2, which is found in the pericellular space in marrow and intracellularly in megakaryocytes.319 Laminin-γ2 chain expression, which is unique to marrow-derived stromal cells, colocalizes with α smooth-muscle actin in marrow and is not expressed in endothelial cells or megakaryocytes.320
Integrins α6β1 and α6β4 are receptors for laminin-10/11 and laminin-8.314 Laminin-10/11 (α5β1γ1/γ5β2γ1) and FN bind CD34+ and CD34+ CD38–progenitors, whereas laminin-8 (α4β1γ1) and laminin-10/11 facilitate CXCL12–mediated transmigration of CD34+ cells.314 In mouse repopulation studies, antibodies that block the α6 components of laminin receptors decreased the homing of HSCs and colony-forming units–granulocyte-macrophage (CFU-GM).321 When combined with antibodies to the α4 component of integrins, antibodies that block the α6 components synergistically decreased marrow homing of the short-term, multipotent repopulating cells. In contrast to this role of these integrin receptors in the homing of HSCs, a 67-kDa, nonintegrin laminin receptor is upregulated in HSCs following G-CSF stimulation and plays a significant role in their mobilization.322 This 67-kDa nonintegrin receptor for laminin also has a role in the marrow homing of burst-forming units–erythroid (BFU-Es), early-stage erythroid progenitors that circulate in the blood.323 On the other hand, the Lutheran blood group glycoproteins serve as receptors for the α5 integrin component of laminins on the late-stage erythroid cells.324 Laminin promotes the M-CSF–dependent proliferation of marrow-derived macrophages via the α6-integrin subunit,325 and α6β1 mediates mast cell adhesion to laminin.326
The TSPs are secreted matrix glycoproteins that modulate cell function by altering cell–matrix interactions.327 TSP1, a multifunctional ECM protein initially identified in platelet α granules, has domains that interact with collagen and FN and may participate in HSC lodgment.328 TSP activates TGF-β329 which results in a stimulatory effect on NK cells.330 TSP binds to matrix heparan sulfates178 and inhibits osteogenic differentiation.331,332 Receptors on hematopoietic and nonhematopoietic cells can interact with TSP, including CD36333 and the cutaneous lymphocyte antigen-1 protein of the CD36/LIMP II gene family.334 CD36 is expressed during erythroid and megakaryocytic maturation.335 TSP stimulates matrix metalloproteinase-9 activity in endothelial cells336 and is chemotactic to monocytes.337 A 140-kDa fragment of TSP1 binds bFGF, and TSP1 acts as a scavenger for matrix-associated angiogenic factors (fibroblast growth factor 2, VEGF, HGF), underscoring its antiangiogenic properties.338,339 Mice deficient in TSP2 demonstrate that TSP2 is taken up in an integrin-dependent manner within the marrow and is necessary for the release of functionally competent platelets by megakaryocytes.340 The 21-amino acid, C-terminal peptide of TSP4 stimulates proliferation of multiple types of early hematopoietic progenitors through the regulator of differentiation 1 (ROD1) nuclear receptor and increases erythropoiesis in mice.341
Vitronectin, a major cytoadhesive glycoprotein, is present in plasma and the interstitial matrix of tissues. It interacts with a vast number of ECM components, cytokines, growth factors and proteolytic enzymes in vitro and in vivo.342 Vitronectin also binds to several αv-containing integrins,342 including the integrin αvβ3 receptor (CD51) on fibroblasts, endothelial cells, osteoclasts,343,344 and mature hematopoietic cells, including megakaryocytes, platelets,345 and mast cells.346 The integrin αvβ3 is expressed on monocyte-macrophages and neutrophils and mediates their transendothelial migration.347,348 The vitronectin receptor cooperates with TSP and CD36 in the recognition and phagocytosis of apoptotic cells by neutrophils, macrophages, and dendritic cells.349,350,351 Vitronectin-deficient mice have normal blood cell counts,352 but thrombogenesis, new microvessel formation and tissue repair capacity are impaired,353 most likely due to failure of inflammatory and thrombotic mechanisms. Thus, in the marrow ECM, vitronectin functions mainly in the coordination of apoptotic cell clearance, cellular migration, bone remodeling, and angiogenesis.
Osteopontin, a glycoprotein produced by osteoblasts and hematopoietic cells in the marrow, binds to FN and collagen.354,355 The predominant form of osteopontin in the marrow is thrombin-cleaved, and its N-terminal peptide is the active ligand for the α9β1 and α4β1 integrins on HSCs and circulating hematopoietic progenitors that plays a role in their attraction to and binding in the marrow.356 Osteopontin can bind numerous integrins and CD44, and its binding through β1-integrin results in suppression of proliferation and maintenance of quiescence in HSCs.354,355 Conversely, the same osteopontin–β1-integrin pathway induces proliferation in erythroblasts.357 Osteopontin also plays a role in the development of NK cells358,359 and T lymphocytes.355 The fibulins are proteins secreted by the stromal cells of marrow, including osteoblasts and endothelial cells.360,361 The metalloproteinase-resistant fibulin-1 accumulates in the ECM where it binds to a specific site on FN,360,361 thereby disrupting HSC binding to FN with resultant decreases in HSC proliferation and differentiation.361 Thus, fibulin-1 can act as a negative regulator that can maintain the quiescence of HSCs in the marrow.
Erythroid progenitor cells arise from MPPs via the activity of the transcription factor GATA-1, which promotes differentiation toward the bipotent MEP that can subsequently differentiate into either erythroblasts or megakaryocytes (Chap. 32).362 MEP fate is determined by the relative activities of two competing transcription factors, KLF-1, which directs differentiation toward the erythroid lineage, and Fli-1, which directs differentiation toward the megakaryocytic lineage.362,363 The earliest progenitor cells committed solely to erythroid differentiation, BFU-Es,364 which are defined by production of large colonies or bursts of erythroblasts after weeks in tissue culture, can circulate in the blood and reenter the marrow. When a BFU-E or one of its progeny, the colony-forming units–erythroid (CFU-Es),364 associates with a marrow macrophage, they form the precursor of the basic unit of terminal erythropoiesis, the erythroblastic island (EBI).94 Under the influence of the KLF-1 in both the macrophage and the erythroid cells,365,366 an EBI develops as a central macrophage surrounded by as many as 30 adherent erythroblasts at various stages of differentiation from CFU-E through enucleating orthochromatic erythroblast. At least five cell-surface protein pairs contribute to adherence between macrophages and erythroblasts in EBIs94: (1) VCAM-1 on macrophages and α4β1 integrin (VLA-4) on erythroblasts; (2) αv component of integrins on macrophages and ICAM-4 on erythroblasts; (3) erythroblast-macrophage protein (EMP), on both erythroblasts and macrophages via a homophilic reaction; (4) CD169/Siglec-1 on macrophages and sialylated glycoproteins on erythroblasts; and (5) hemoglobin-haptoglobin receptor (CD163) on macrophages and an unknown binding partner on erythroblasts.
Differentiating erythroblasts are defined as basophilic, polychromatophilic, and orthochromatic erythroblasts by their morphologic appearances in Giemsa-stained films of aspirated marrows. However, CFU-Es and their immediate progeny, the proerythroblasts (ProEBs), as well as the morphologically defined, later erythroblast stages can be purified and defined by flow cytometry. Murine erythroid cells from CFU-Es through ProEBs, are identified by flow cytometric expression patterns of transferrin receptor (CD71) and the erythroid-specific membrane glycoprotein Ter119,367 or of CD44 and forward light scatter.368 Likewise, expression patterns of glycophorin A, Band 3, and the α4 component of integrin permit identification of the same stages in human erythroid differentiation.369
In EBIs, CFU-Es lose SCF dependence that had been present throughout their differentiation from HSCs, and CFU-Es, ProEBs, and early basophilic erythroblasts develop a dependence upon EPO to prevent apoptosis.370 The level of EPO, the principal regulator of erythropoiesis, is regulated by tissue oxygen delivery in the kidney, and is dependent on both blood oxygen levels and red cell numbers.370 However, during hypoxic stress, CFU-Es and ProEBs can be increased without differentiation in response to circulating glucocorticoid hormones371,372 and BMP4 from central macrophages of EBIs.373 EPO prevents apoptosis by decreasing expression of Fas, a membrane protein of the TNF-α receptor family that is prominently expressed on CFU-E, ProEBs and early basophilic stage erythroblasts. Fas activation triggers a series of caspases, a family of intracellular proteases that cleave other caspase members in sequential fashion, ultimately inducing apoptosis.374 Fas-ligand, which binds and activates Fas, is produced mainly by immature erythroblasts in mice375 and by mature erythroblasts in humans.376 EPO also suppresses apoptosis in late-stage erythroblasts by inducing the antiapoptotic protein Bcl-XL, which stabilizes mitochondria, preventing the activation of caspases other than those activated by Fas.377 As a result of the Fas/Fas-ligand negative feedback within the EBI, differentiating erythroblasts can modulate the rate of CFU-E/ProEB apoptosis and provide regulated control rates of erythrocyte production commensurate with erythropoietic demand.
In EBIs, differentiation events include: (1) hemoglobin production in differentiating erythroblasts, (2) formation of the erythrocyte plasma membrane and underlying membrane skeleton, (3) cell size decrease associated with the terminal 4 to 5 cell divisions being a result of decreased duration of the G1 phase of erythroblasts attached to central macrophages,378 and (4) nuclear condensation,379 stiffening,380 and extrusion.381 Erythroblast enucleation requires nonmuscle myosin IIB382 and filamentous actin381 to produce a membrane-enveloped nucleus and a nascent reticulocyte. The central macrophage sends out extensive slender membranous processes that envelop each erythroblast and phagocytize defective erythroblasts and extruded nuclei.383 The extruded nuclei display phosphatidylserine on their plasma membranes that leads to rapid phagocytosis by the central macrophage.384 Phagocytosis of extruded nuclei with recycling of the DNA components is essential in that deoxyribonuclease II–deficient mice die from an underproduction anemia with fetal liver macrophages filled with extruded erythroid nuclei.385 The irregularly shaped, maturing reticulocytes can interact directly with the central macrophages before entering the blood through the venous sinuses.94
During thrombopoiesis, HSC in the subcortical regions of the hematopoietic cords generate megakaryocytes by sequential, overlapping expressions of specific transcription factors. First HSCs differentiate to common myeloid progenitors (CMPs) via the influence of PU.1 and GATA-1, next to MEPs via GATA-1/FOG, then to megakaryocytic progenitors via Fli-1, and finally to megakaryocytes via NF-E2 (Chap. 111).362,386 The microenvironmental factors that control survival and differentiation of megakaryocytes and their progenitors include a similar pattern of dependence to that of erythroid progenitors, with an overlapping decrease in dependence on SCF and an increasing dependence upon a physiologically regulated cytokine, TPO in the case of megakaryocytes, which ceases before the completion of differentiation.386,387 TPO concentrations are reciprocally related to the circulating platelet mass, which is the major site of metabolism of the hormone.388 As the major regulator of megakaryocyte development, TPO acts in concert with several synergistic cytokines, including IL-11, IL-3, and IL-6.386,387 TPO induces endomitosis in terminally differentiating megakaryocytes by inhibiting cytokinesis through reduced function of the contractile ring of filamentous actin and suppression of nonmuscle myosin expression.389,390 However, DNA replication and accumulation of cytoplasmic proteins continues during six to seven of these endomitotic cell cycles. The resultant polyploid nucleus and abundant cytoplasm characterize the mature megakaryocyte which can account for 2 percent of marrow hematopoietic cell volume.93
Mature megakaryocytes lie directly outside the marrow vascular sinus wall391
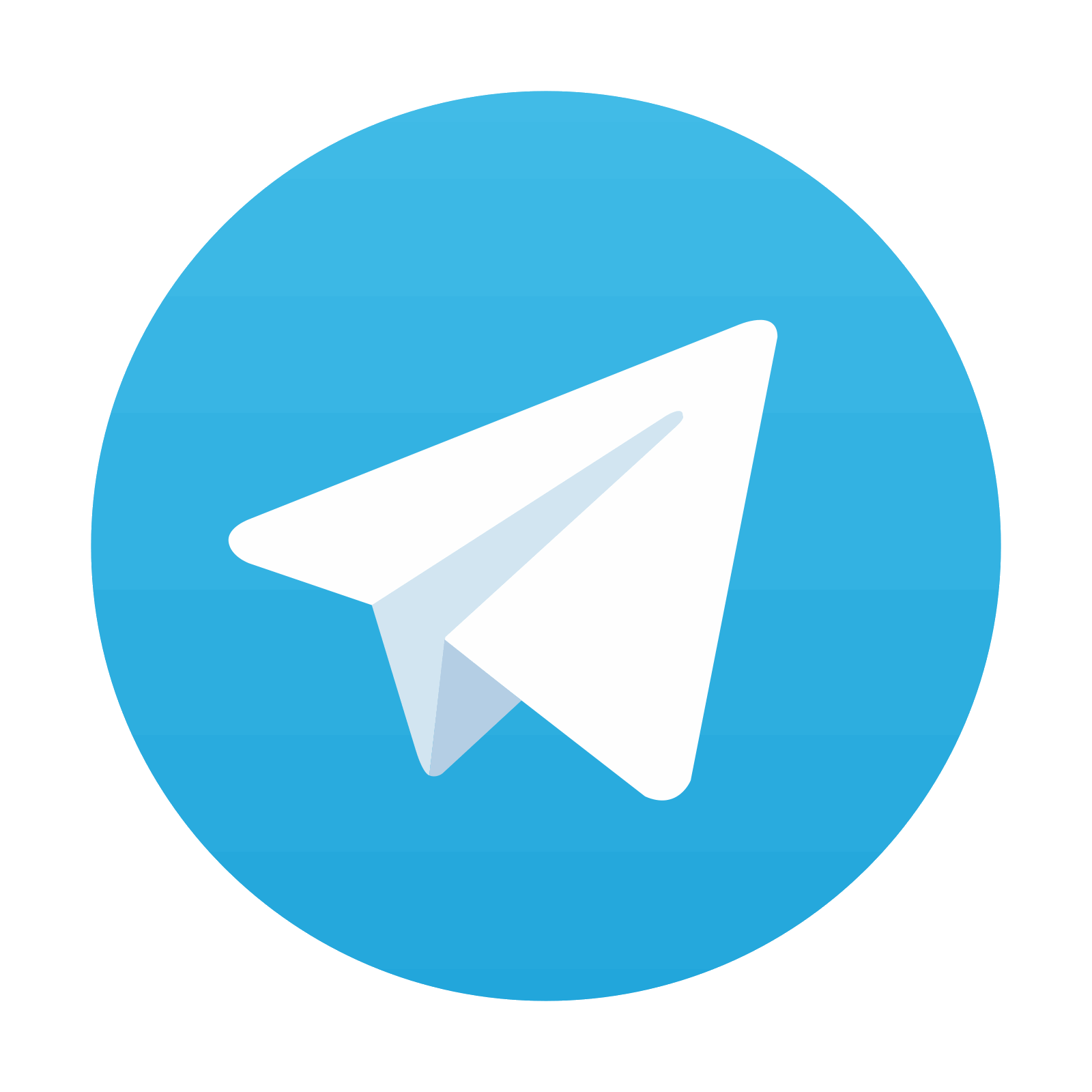
Stay updated, free articles. Join our Telegram channel
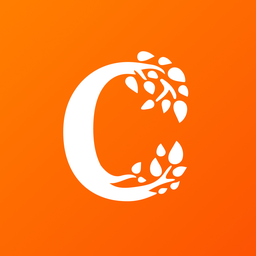
Full access? Get Clinical Tree
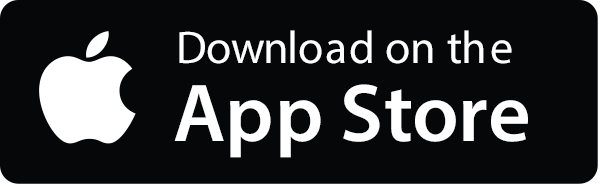
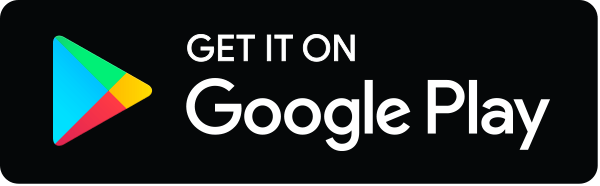
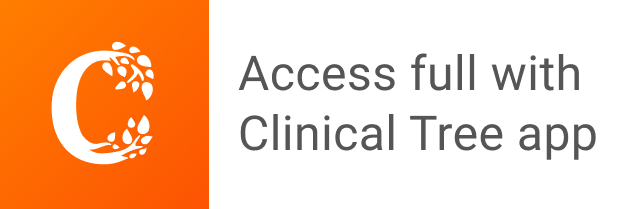