Martin Dreyling and Michael E. Williams (eds.)Hematologic MalignanciesRare Lymphomas201410.1007/978-3-642-39590-1_4
© Springer-Verlag Berlin Heidelberg 2014
4. Signaling Pathways in Rare Lymphomas
Andrew Lipsky1, Patricia Pérez-Galán3, Claudio Agostinelli4, Pier Paolo Piccaluga4, Stefano A. Pileri4 and Adrian Wiestner2
(1)
Department of Internal Medicine, Montefiore Medical Center, Albert Einstein College of Medicine, New York, NY, USA
(2)
Hematology Branch, National Heart, Lung, and Blood Institute, National Institutes of Health, Bethesda, MD, USA
(3)
Department of Hemato-Oncology, Institut d’Investigacions Biomédiques August Pi I Sunyer (IDIBAPS), Barcelona, Spain
(4)
Pathology and Haematopathology Unit, Department of Specialty, Diagnostic and Experimental Medicine, Bologna University School of Medicine, Bologna, Italy
4.1.1 Cell Cycle Deregulation
4.1.2 DNA Damage Pathway
4.1.3 B-Cell Receptor Pathway
4.1.4 PI3K/AKT/mTOR Pathway
4.1.5 JAK/STAT Pathway
4.1.6 NOTCH Pathway
4.2.1.1 JAK/STAT Pathway
4.2.1.2 NF-κB Pathway
4.2.2 Burkitt’s Lymphoma
4.2.2.2 BL and the INK4a/ARF Network
4.2.3 MALT Lymphoma
4.2.3.1 NF-κB Pathway
4.2.4.2 MYD88 and Oncogene Addiction
4.3.1 Histogenesis
4.3.2 Pathogenetic Mechanisms
Abstract
Here we give an overview of select signaling pathways understood or thought to play a key role in the pathogenesis of lymphomas, covering examples of both B-cell and T-cell lymphomas. The selection focuses on providing well-understood examples and may serve as a guideline also for entities not covered in these sections. Remarkably many of these cancers are characterized by genetic alterations that lead to activation of the NF-κB and JAK/STAT pathways. A striking example is seen in MALT lymphoma where recurrent chromosomal translocations targeting different regulatory nodes lead to NF-κB activation. We discuss these mechanisms as well as more disease-specific alterations such as oncogenic activation of MYC in Burkitt’s lymphoma, of TAX in adult T-cell leukemia/lymphoma, of ALK in peripheral T-cell lymphoma, and the MyD88 L265P mutation in Waldenström’s macroglobulinemia. DNA damage pathways are equally of importance and discussed in the context of with mantle cell lymphoma and Burkitt’s lymphoma. A striking example of how dysregulation of immune surveillance pathways interfaces with activation of oncogenic pathways is seen in the case of primary mediastinal B-cell lymphoma.
4.1 MCL, a Paradigm of Multiple Activated Pathways in a Mature B-Cell Lymphoma
Mantle cell lymphoma (MCL), a mature B-cell neoplasm defined as a distinct entity in the early 1990s, constitutes about 6 % of all non-Hodgkin’s lymphomas (NHL) (O’Connor 2007; Dreyling and Hiddemann 2009; Ghielmini and Zucca 2009; Jares et al. 2007). MCL is typically disseminated at presentation, with a leukemic component in 20–30 % of patients. Classic and blastoid variants are recognized, the latter associated with inferior clinical outcome. MCL is one of the most difficult to treat B-cell lymphomas. While conventional chemotherapy induces high remission rates in previously untreated patients, relapse within a few years is common, contributing to a rather short median survival of 5–7 years (Herrmann et al. 2009; Martin et al. 2008). MCL is a complex disease, where several deregulated pathways contribute to its aggressiveness. Here we described those pathways that have been found to play a key role in MCL pathogenesis.
4.1.1 Cell Cycle Deregulation
The genetic hallmark of MCL is the translocation t(11;14)(q13;q32) which leads to constitutively high expression of cyclin D1. Additional mutations in the cyclin D1 transcript delete regulatory elements that normally shorten mRNA half-life. These mutations further increase cyclin D1 expression (Rosenwald et al. 2003). However, cyclin D1-negative cases having typical morphology and gene expression profile have been described and often show overexpression of cyclin D2 or D3 (Rosenwald et al. 2003). Cyclin D1 is labile protein of 30 kDa that forms a complex with the cyclin-dependent kinase CDK4 or CDK6 to promote cell cycle entry. The CDK4 locus is frequently amplified (Bea et al. 1999), and decreased expression of miR-29, which targets CDK6, can lead to increased CDK6 expression and identifies patients with short survival (Zhao et al. 2010). Transcription, translation, assembly into holoenzyme complexes, subcellular localization, and degradation of cyclin D1 are tightly regulated. While overexpression of cyclin D1 is not transforming in nude mice, additional events that increase nuclear cyclin D1 levels and the activity of signaling pathways that regulate D1 translation and protein stability can enhance its oncogenic potential.
4.1.2 DNA Damage Pathway
MCL shows genetic alterations that affect DNA damage response pathways and are of particular interest because they may contribute to refractoriness to chemotherapy (Bea et al. 2009). 11q22–23 deletions affecting the ATM gene are recurrent in MCL. The ATM kinase is critically involved in the cellular response to DNA damage and may act as a tumor suppressor gene. Truncating or missense mutations involving the PI3K domain of ATM are found in a majority of MCL cases and are commonly accompanied by the loss of the other allele. The high frequency of ATM mutations in MCL is striking and has been linked to ATM expression in naïve B cells in the mantle zone. Another mechanism for the strong selective pressure on ATM mutant clones may be aberrant re-initiation of DNA replication during S-phase leading to double-strand DNA breaks and activation of the ATM pathway (Kim and Diehl 2009). Moreover, the tumor suppressor gene TP53, downstream of ATM, plays an important role in DNA damage responses. Mutations of TP53 typically in conjunction with 17p13 deletions have been detected primarily in blastoid MCL cases. In addition, UPDs involving the chromosomal band 17p are associated with TP53 inactivation. An alternative mechanism to disrupt the p53 pathway involves overexpression of the negative regulators MDM2 and MDM4. MDM2 overexpression due to copy number gains correlates with inferior survival. Similarly, MDM4, which is also highly expressed in MCL, decreases expression of the CDK inhibitor p21, thereby promoting cell cycle progression (Liang et al. 2010).
4.1.3 B-Cell Receptor Pathway
Recent studies reported constitutive activation of the B-cell receptor (BCR) signal transduction components SYK and PKCβII (Boyd et al. 2009; Rinaldi et al. 2006). SYK was amplified in both Jeko-1 cells and some primary MCL samples, but constitutive activity of SYK was only demonstrated in the cell line (Rinaldi et al. 2006). Jeko-1 cells were more sensitive to a SYK inhibitor than MCL cell lines without constitutive SYK activation, indicating some dependence on the pathway. In a screen for phosphoproteins, PKCβII was found to be phosphorylated in primary MCL samples in contrast to normal B cells (Boyd et al. 2009). However, inhibitors targeting these molecules have induced only minor clinical responses. On the contrary, targeting the downstream kinase BTK (Bruton’s tyrosine kinase) has yielded objective responses in a few patients with MCL (Advani et al. 2010). These results may suggest a role for BCR signaling in MCL pathogenesis.
4.1.4 PI3K/AKT/mTOR Pathway
The PI3K/AKT pathway is involved in the transduction of a variety of extracellular signals and plays a prominent role in many cancers (Engelman 2009). In normal B cells, PI3K functions as a transducer of BCR signaling that regulates proliferation, differentiation, apoptosis, and survival. Gene expression profiling implicated the PI3K/AKT pathway in the pathogenesis of MCL (Rizzatti et al. 2005), and several key components of the PI3K/AKT/mTOR pathway are activated in MCL (Peponi et al. 2006), indicating a possible contribution of this pathway to MCL pathogenesis. Constitutive activation of AKT was found in most blastoid and many classic MCL tumors and was associated with the phosphorylation of downstream targets including MDM2, Bad, and p27 (Dal Col et al. 2008; Rudelius et al. 2006). Furthermore, AKT-mediated activation of mTOR and its downstream targets S6K and eukaryotic initiation factor 4E-binding protein-1 (4E-BP1) can increase translation of key proteins.
Several mechanisms may cause constitutive activation of AKT, including activation of upstream kinases such as SYK, and amplification of PI3KCA, the gene encoding the catalytic subunit p110α (Psyrri et al. 2009).In contrast to solid tumors, no activating somatic mutations of PI3KCA have been identified (Rudelius et al. 2006; Psyrri et al. 2009). Loss of PTEN, a phosphatase that turns the PI3K pathway off, is another recurrent feature in MCL and may be the result of mutations, deletions, or promoter methylation (Rudelius et al. 2006). PTEN can also be inactivated by phosphorylation at Ser380 and Thr382/383, which has been found in MCL cases with constitutively active AKT (Dal Col et al. 2008).
4.1.5 JAK/STAT Pathway
The JAK/STAT signaling pathway regulates growth, proliferation, differentiation, and survival in response to external stimuli especially cytokines (Sun et al. 2004). The JAK/ STAT pathway is aberrantly activated in several B-cell lymphomas, including in primary mediastinal B-cell lymphoma (discussed in the next section). In MCL, the active, phosphorylated form of STAT-3 was found in 47 % of nodal cases (Kawadler et al. 2008) and in 70 % of leukemic cases (Düwel et al. 2009). It has been hypothesized that STAT-3 activation may be through activation of the BCR and/or interleukins 6 and 10 (Düwel et al. 2009).
4.1.6 NOTCH Pathway
NOTCH receptors play a critical role in cell fate specification during development and participate in multiple biological processes. In the hematopoietic system, NOTCH1 activation plays a critical role at multiple stages in both T- and B-cell development (Pui et al. 1999). The NOTCH1 receptor functions as a ligand-activated transcription factor that directly transduces extracellular signals in the cell surface into changes in gene expression in the nucleus. Activation of NOTCH receptors typically occurs via cell-cell contact and interaction of a NOTCH protein with a delta-like or jagged ligand expressed on the surface of a neighboring cell.
Whole transcriptome sequencing in MCL samples uncovered recurrent somatic mutations in NOTCH1 coding sequence in 12 % of clinical samples and 20 % of cell lines. These mutations generate a premature stop codon, resulting in a NOTCH1 protein lacking the C-terminal domain, which contains a PEST sequence (a sequence rich in proline, glutamic acid, serine, and threonine). Removal of this region results in the accumulation of an active protein isoform. NOTCH1 mutations were associated with poor overall survival (Kridel et al. 2012). The pattern and frequency of NOTCH1 mutations is very similar to what has recently been described in chronic lymphocytic leukemia (CLL) (Puente et al. 2011) but different from T-cell acute lymphoblastic leukemia (T-ALL) (Paganin and Ferrando 2011), where NOTCH1 mutations arise in more than 50 % of cases and target the heterodimerization and/or the PEST domains of NOTCH1.
4.2 Signaling Pathways in Other B-Cell Lymphomas
4.2.1 Primary Mediastinal B-Cell Lymphoma
Primary mediastinal B-cell lymphoma (PMBL) represents 2–4 % of NHL and since 2001 is considered a separate clinicopathologic entity, different from diffuse large B-cell lymphoma (DLBCL) and characterized by a distinct gene expression profile (Lenz et al. 2008). PBML often presents with a bulky tumor in the anterior mediastinum and progresses rapidly, affecting primarily women in the third or fourth decade. PBML shows similarities with classic Hodgkin lymphoma in terms of genetic alterations and gene expression profiling. In fact, both lymphomas show activation of Janus kinase-signal transducer and activator of transcription (JAK-STAT) and nuclear factor-kB (NF-kB) signaling pathways that increase proliferation and survival of tumor cells. However, PBML also has the ability to bypass the immune surveillance, adding another level of complexity and an advantage for the malignant clone.
4.2.1.1 JAK/STAT Pathway
A common genetic lesion in PBML present in more than half of cases is gain of chromosomal region 9p, that involves among other genes, JAK2 (Lenz et al. 2008; Wessendorf et al. 2007). Additional alterations further support the importance of this pathway in PBML pathogenesis. Gene expression studies have demonstrated overexpression of JAK2 in PMBL and have suggested constitutive activation of the IL-4 and IL-13 pathways as a result (Savage et al. 2003). Accordingly, STAT6, the transcription factor that is primarily regulated by IL-4 and IL-13, is constitutively activated in PMBL, and somatic mutations in the DNA-binding domain of STAT6 have been found in 36 % of PMBCL cases (Guiter et al. 2004; Ritz et al. 2009). Surprisingly, despite JAK2 amplification and an increase in JAK2 mRNA, only minimal changes in JAK2 protein have been documented in PMBL cell lines. However, a clear prolongation of the JAK2 protein half-life was observed, resulting in decreased protein turnover (Melzner et al. 2006). Moreover, a negative regulator of the JAK/STAT pathway, Src-homology 2 domain containing suppressor of cytokine signaling 1 (SOCS1), is frequently deleted in PMBL. SOCS1 targets JAK for proteasomal degradation. SOCS1 is deleted in up to 45 % of cases making it the most common recurrently mutated tumor suppressor gene in PMBL. Biallelic deletions of SOCS1 have also been detected in PMBL cell lines (Melzner et al. 2005).
4.2.1.2 NF-κB Pathway
Mutations and structural alterations of genes belonging NF-κB have been described in PBML leading to constitutive NF-κB activity. Frequent gains (~50–75 % of cases) of 2p14–16 affecting the REL proto-oncogene are present in PBML, and nuclear expression of this transcription factor has been described (Bentz et al. 2001; Joos et al. 1996; Weniger et al. 2007). In addition, gene expression profiling studies have demonstrated increased expression of a NF-κB gene signature expressed in PMBL, and a possible role for TNF-α signaling has been entertained. (Feuerhake et al. 2005) Another gene at this chromosomal location is the zinc finger transcriptional repressor BCL11A that encodes a protein critical to lymphoid development. BCL11A is thought to be the oncogene providing a selective advantage to cells harboring the 2p amplification. BCL11A is present at increased copy numbers in 75 % of cases, and high nuclear protein expression is seen in 88 % of cases. This suggests that BCL11A-dependent transcriptional repression may provide a survival advantage to the malignant B cell (Weniger et al. 2006). Other chromosomal imbalances affecting NF-κB-related proteins are amplification of BCL10 (1p22) and MALT1 (18q21). Recently, TNFAIP3 (encoding A20) has been reported as a novel tumor suppressor gene in PMBL. The A20 protein acts as a ubiquitin-modifying enzyme that inhibits NF-κB signaling downstream of TNF receptor engagement by interacting with RIP1, TRAF1, and TRAF2. Mutations in TNFAIP3 leading to constitutive NF-κB activity were found in 36 % of PMBCL cases (Schmitz et al. 2009; Honma et al. 2009).
4.2.1.3 Dysregulation of Immune Surveillance Pathways
Histologically, PMLBL includes variable numbers of malignant cells within an inflammatory infiltrate, suggesting that these tumors escape immune surveillance and that this immune privilege may contribute to the cancer phenotype. To date, two groups of molecules have been identified as responsible of this privileged microenvironment: MHCII and PD ligands.
Early studies recognized that PBML shows reduced expression of major histocompatibility complex class II(MHC II) genes in a substantial number of cases (Moller et al. 1986). Subsequently, this decreased expression of MHCII was found to be associated with inferior survival (Roberts et al. 2006). Moreover, in a large series 65 % of PBML cases showed decreased MHCII expression which correlated with reduced numbers of cytotoxic T lymphocytes (Farinha et al. 2009). These data indicate a possible immune escape through downregulation of HLA class II molecules. Interestingly, loss of MHCII expression, at least in part, may be due to unbalanced genomic rearrangements of 16p13.13 affecting CIITA, a positive regulator of MHCII expression (Steidl et al. 2011). Of note, the SOCS1 gene, which is frequently deleted in PBML, resides close to CIITA on chromosome 16.
PDL1/CD274 and PDL2/CD273 belong to the CD28 superfamily of costimulatory receptors that regulate T-cell activation. PDL1 and PDL2 serve as negative regulators of CD8+ T-cell activation and proliferation. Gene expression profiling studies revealed PDL2/CD273 overexpression in PMBL. The genes encoding PDL1 and PDL2 are located at chromosome 9p, where gains have been found in more than half of PBML cases. Studies of copy number and mRNA expression confirmed simultaneous overexpression of JAK2 and the PD ligands in cases with 9p amplification and demonstrated that JAK2 further augments their expression through increased transcription. In fact, a direct correlation between 9p copy number changes, and mRNA expression of both PD ligands has been demonstrated (Green et al. 2010). In addition, both PDL1/CD274 and PDL2/CD273 have been found as recurrent gene fusion partners of CIITA. As a result of the translocation t(9;16)(p24.1;p13.13), both PDL1 and PDL2 are highly expressed under control of CIITA promoter III. Thus, overexpression of PD ligands could further contribute to immune evasion. Indeed, in vitro studies showed that U2940, a PMBL cell line expressing high wild-type levels of PD ligands, induced energy in cocultured Jurkat T cells.
Interestingly, detailed cytogenetic studies of the translocated CIITA locus at 16p13.13 found that a substantial number of cases harbor additional unbalanced rearrangements with loss of genetic material centromeric of the breakpoint. This finding is in keeping with previous reports describing deletions of the tumor suppressor gene SOCS1 located in the vicinity of CIITA on chromosome 16 (Melzner et al. 2006). Thus, a single genetic event may trigger a complex genetic rearrangement that simultaneously contributes to immune evasion (MHCII downregulation as a consequence of CIITA disruption and inhibition of T-cell activation through upregulation of PD ligands) as well as activation of growth and survival pathways (JAK2 amplification and deletion of the negative regulator SOCS1).
4.2.2 Burkitt’s Lymphoma
Burkitt’s lymphoma (BL) is a mature aggressive B-cell NHL (Dalla-Favera et al. 1982). BL occurs in three distinct clinical varieties: an endemic variant, arising in children in equatorial Africa (coinciding with areas of malaria endemicity) that is often associated with Epstein-Barr virus (EBV); a sporadic variant, observed in adolescents and young adults in Europe and North America that is less frequently associated with EBV; and an immunodeficiency-related variant, most commonly observed in HIV-infected individuals (Swerdlow et al. 2008; Molyneux et al. 2012). In high-risk areas endemic Burkitt’s lymphoma has an incidence of 4–5 per 100,000 children, whereas in lower-risk areas the incidence of sporadic BL is about tenfold less (Cardy and Sharp 2001; Rainey et al. 2007). Each of the three variants is characterized by a chromosomal rearrangement resulting in the dysregulation and overexpression of the MCY proto-oncogene (Thorley-Lawson and Allday 2008). MCY encodes a basic helix-loop-helix (bHLH) transcription factor that is involved in regulating a diverse set of >1,500 downstream genes (Zeller et al. 2006). By forming a heterodimer with its bHLH partner protein Max, MCY exerts direct or indirect regulatory influence on 15 % of the genome including genes that play a role in cell cycle regulation, growth, protein biosynthesis, and apoptosis (Dang et al. 2006; Hecht and Aster 2000). In fact, MCY is thought to be a global transcriptional activator through its role in modulating chromatin structure (Klapproth and Wirth 2010). Recent work has demonstrated that in addition to regulating transcription, MCY can regulate microRNA networks that plays a role in the modulation of tumorigenesis (Thorley-Lawson and Allday 2008; Chang et al. 2007; Sander et al. 2009). The malignant B-cell clone expresses CD19, CD20, and low to intermediate levels of CD10. As one of the fastest growing human tumors, >95 % of tumor cells express Ki-67 (Hecht and Aster 2000). On tissue microscopy, BL is further characterized by the classical “starry sky” appearance which is produced by numerous apoptotic cells interspersed with pale macrophages. Though there remains some controversy, observations based in part on the patterns and rates of somatic hypermutations in the variable region of the immunoglobulin chain suggest that the likely precursor cell of origin is the germinal center B cell in the case of EBV-negative BL and the memory B cell in the setting of EBV-positive BL (Thorley-Lawson and Allday 2008; Hochberg et al. 2004).
Several translocations are commonly observed in BL which associate MYC to one of three immunoglobulin loci. The most prominent translocation, t(8:14)(q24;q32), has been observed in 85 % of all BL, including both EBV positive and negative forms of disease (Yustein and Dang 2007; Zech et al. 1976). It is associated with the juxtaposition of MYC and the enhancer element of the Ig heavy chain. Other translocations including t(2;8)(p12;q24) and t(8;22)(q24;q11) occur in 10–15 % of patients and involve the kappa and lambda light chains, respectively (Molyneux et al. 2012; Kornblau et al. 1991). While additional chromosomal abnormalities are detected in up 70 % of pediatric BL, the total number of such additional genetic events in any one case is relatively low (Salaverria et al. 2008). It has been known for some time that trisomies of chromosomes 7, 8, 12, and 18 are frequent, and more recent results from whole genome mapping have allowed for identification of recurrent aberrations at other loci.
4.2.2.1 MYC Expression, p53, and Apoptosis
Paradoxically, elevated expression of MCY has been demonstrated to induce apoptosis; (Hoffman and Liebermann 2008) this is accomplished via upregulation and expression of pro-apoptotic genes including TP53 and ARF1, as well as downregulation or disruption of anti-apoptotic genes such as BCL2 and BCL2L1 (Klapproth and Wirth 2010; Meyer and Penn 2008). The prevailing explanation of this observation is that apoptosis serves as a “built-in” failsafe, balancing any unrestrained growth resulting from aberrant MYC activation. Tumorigenesis is thought to result when this failsafe is circumvented. Indeed, TP53 mutation is observed in the majority of BL cell lines and at least 30 % of BL tissue biopsies; thus, MCY activation and proliferation may select for TP53 inactivation via point mutation (Lindström and Wiman 2002). Additional MCY-dependent mechanisms may also contribute to tumorigenesis. For example, MCY can increase expression of cyclin D and E, thereby dysregulating the phosphorylation of pRB, leading to promotion of the G1 to S cell cycle transition (Lindström and Wiman 2002; Santoni-Rugiu et al. 2000). Also, MCY may directly repress the transcription of cell cycle inhibitors including p27 and p21 (Chandramohan et al. 2008; Gartel et al. 2001).
4.2.2.2 BL and the INK4a/ARF Network
The INK4a-ARF locus encodes two potent tumor suppressors, p16Ink4a and p14ARF, which contribute to the regulation of RB and p53 (Lowe and Sherr 2003). RB acts to prevent entry into the S phase of the cell cycle by suppressing E2F transcription factors responsible for activation of several genes involved in DNA replication (Sherr 2001). Phosphorylation of RB during the G1 phase is catalyzed by cyclin D and E resulting in the loss of RB’s ability to suppress E2Fs. The Ink4 proteins, including the canonical protein P16INK4a, inhibit cyclin D-dependent kinases (CDK4 and CDK6), thereby maintaining RB-E2F repression (Lowe and Sherr 2003). Interestingly, MYC activation has been shown to activate cdc25A, a CDK-activating phosphatase that acts on CDK4 and CDK6, a process which might induce increased RB phosphorylation (Galaktionov et al. 1996). In addition, silencing of the p16/INK4a gene via methylation is commonly observed, occurring in 89 % of BL cell lines and 42 % of primary biopsies (Klangby et al. 1998). Interestingly, p73, a gene located on 1p36.2–3 that is functionally homologous to p53, has been shown to also undergo aberrant promoter methylation in 30 % of BL (Corn et al. 1999). Additionally, in a subset of BL cells that express wild-type p53, inactivation of p53 is mediated by MDM2 that targets of p53 for proteasomal degradation (Lindström and Wiman 2002; Capoulade et al. 1998). Therefore, in a subset of BL, inactivation of the ARF-MDM2-p53 pathway is thought to allow the escape from MCY-induced apoptosis early in BL development (Lindström et al. 2001). This conclusion was reinforced by the observation that the ARF-MDM2-p53 apoptotic pathway is disrupted in approximately 55 % of sporadic BL (Wilda et al. 2003).
4.2.2.3 Additional Genetic Abnormalities and Cofactors
Most recently somatic mutations in the transcription factor TCF3 (E2A) or its negative regulator ID3 have been described in 70 % of BL cases (Schmitz et al. 2012). TCF3 activates the pro-survival PI3K pathway in BL, in part by augmenting tonic B-cell receptor signaling. In addition, mutations of CCND3 were found in 38 % of sporadic BL cases, and were shown to result in the production of highly stable cyclin D3 isoforms that mediate cell cycle progression.
The BCL6 proto-oncogene, known to be implicated in chromosomal translocations in diffuse large-cell lymphomas, has also recently been shown to undergo mutations in the setting of BL (Capello et al. 2000). In one study, the frequency of aberration was detected to be 28.6 % in sporadic BL and 50 % in endemic BL (Capello et al. 1997). A mechanism for accrual of BCL-6 mutation similar to that of somatic hypermutation of IG genes is suggested; this accords well with both the observation that BCL-6 mutations arise during transit through the germinal center and the notion that BL arises from germinal or post-germinal center B cells (Capello et al. 2000).
Several cofactors are also thought to play an important role in BL. In the endemic variety, infection with Plasmodium falciparum is thought to contribute to BL pathogenesis via reactivation of EBV through the malaria parasite’s cysteine-rich interdomain 1a (Donati et al. 2004). The induction of TLR-9 signaling via ligand activation is also implicated in pathogenesis, as in human B cells, it can induce cytidine deaminase, a key enzyme involved in chromosomal translocations. Despite the fact that several observations imply a link between EBV and tumorigenesis, the exact mechanism is controversial and remains to be elucidated. The prevailing assumption is that EBV plays a role in fixing genetic and epigenetic changes that either inhibit apoptosis directly or shield BL cells from the growth constrains established by the immune system and the tumor microenvironment (Gruhne et al. 2009).
4.2.3 MALT Lymphoma
Extranodal marginal zone B-cell lymphoma of mucosa-associated lymphoid tissue (MALT lymphoma) is an extranodal lymphoma that occurs in a wide variety of sites including the stomach, lung, salivary gland, thyroid, and small bowel. Clinically, MALT lymphoma may be classified as low or high grade and presents in adults (with a median age of 61 years), the majority of whom exhibit localized disease (Isaacson 2005). Most cases originate in the gastric mucosa and are strongly associated with chronic Helicobacter pylori infection. Accounting for approximately 7 % of all NHLs and 50 % of gastric lymphomas, MALT lymphoma is the most common extranodal lymphoma and is characterized by the cytological features and immunophenotype of marginal zone B cells, including expression of CD19, CD20, and CD22 and negativity of CD5, CD10, and CD23 (Isaacson 2005; Zucca et al. 2000).
4.2.3.1 NF-κB Pathway
Karyotype studies of MALT lymphoma have revealed four recurrent and mutually exclusive chromosomal aberrations implicated in its pathogenesis: t(11;18)(q21;q21), t(1;14)(p22;q32), t(14;18)(q32;q21), and t(3;14)(p13;q32) (Du 2011). All four translocations promote activation of NF-κB activation. This effect is mediated via the uncoupling of the BCL-10/MALT1 signaling complex from physiologic upstream stimuli (Lucas et al. 2001).
Under physiologic conditions, activation of the canonical NF-κB pathway is initiated when antigen-receptor stimulation triggers the recruitment of the scaffolding adaptor CARMA1 (CARD11), phosphorylating its protein kinase C (PKC)-regulated domain (Rawlings et al. 2006). A conformational change in CARMA1 allows for recruitment of BCL10 and triggers a subsequent oligomerization cascade. Oligomerized BCL10 then induces oligomerization of MALT1 by binding to its immunoglobulin (Ig)-like domain, forming the CARMA1 complex. This CARMA1 complex directly interacts with TRAF6, conferring ubiquitin-ligase activity via the oligomerization of TRAF6 by oligomerized MALT1. The interaction of TRAF6 with the ubiquitin-conjugating enzyme E2 facilitates the K-63-linked polyubiquitylation of IκB kinase-γ (IKKγ) [alternatively designated nuclear factor-κB essential modulator (NEMO)]. An activated IκB kinase (IKK) complex results when ubiquitylated IKKγ, acting as the regulatory subunit of the complex, activates IKKα and IKKβ, the two catalytic subunits (Du 2011). The activated IKK complex phosphorylates IκB, resulting in its proteolytic degradation by the 26S proteasome. NF-κB dimers are thereby released and translocated to the nucleus where they upregulate genes involved in cellular activation, proliferation, and survival.
The translocation t(1;14)(p22;q32) found in 5 % of MALT lymphomas results in the overexpression of BCL10 by placing the whole BCL10 gene under the regulatory control of the IG gene enhancer (Du 2011; Willis et al. 1999; Zhang et al. 1999). As mentioned above, BCL10 is expressed primarily in the cytoplasm of normal lymphocytes where it functions as an adaptor protein, coupling the protein CARMA1 to MALT1. This effect is mediated upstream via CARD-CARD (caspase recruitment domain) interactions and downstream via BCL10 interaction with two N-terminal immunoglobulin-like domains on MALT1 (Lucas et al. 2001). In the setting of BCL10 overexpression and in the absence of upstream signaling, the N-terminal CARD facilitates the formation of BCL10 oligomers, leading to MALT1 oligomerization and constitutive NF-κB activation. Furthermore, recent work suggests that BCL10 may also play a role in activation of the noncanonical NF-κB pathway (Du 2011; Bhattacharyya et al. 2010).
The translocation (11;18)(q21;q21) causes fusion of the apoptosis inhibitor-2 gene (which inhibits the biological activity of caspases 3, 7, and 9) with the MALT1 protein (Isaacson and Du 2004). The action of this API2-MALT1 fusion protein is sufficient for NF-κB activation, a property that is not independently exhibited by either wild-type API2 or MALT1 (Lucas et al. 2001; Isaacson and Du 2004). The API2 gene contains three N-terminal baculovirus inhibitor of apoptosis repeats (BIR) that may mediate the oligomerization of the API2-MALT1 fusion protein, which when accompanied by TRAF2 binding renders the protein capable of NF-κB activation (Garrison et al. 2009).
The translocation t(14;18)(q32;q21) places the MALT1 gene under the control of the enhancer region of the Ig-heavy-chain gene leading to dysregulation and overexpression of MALT1 (Sanchez-Izquierdo et al. 2003; Streubel et al. 2003). The paracaspase MALT1 has several known points of intersection with the NF-κB pathway. First, it plays a part in the aforementioned oligomerization cascade: after self-oligomerization, activated MALT1 binds to TRAF6 inducing its oligomerization and subsequent IKK complex activation (Sun et al. 2004). Second, MALT1 may activate the NF-κB pathway through heterodimerization and activation of caspase-8, a caspase required for lymphocyte proliferation (Kawadler et al. 2008). Additionally, through its protease activity and attenuation of the global NF-κB inhibitor A20, MALT1 also regulates NF-κB activation, although such protease activity is not essential for IκB phosphorylation (Du 2011; Düwel et al. 2009).
Recently, a translocation (3;14)(p13;q32) juxtaposing the immunoglobulin gene heavy-chain enhancer and the forkhead box protein P1(FOXP1) at 3p14.1 results in FOXP1 dysregulation (Streubel et al. 2005). Expressed in normal and neoplastic B cells, FOXP1 has been shown to be highly expressed in a subset of diffuse large B-cell lymphomas, and suspicion that it may regulate NF-κB activation has been bolstered by the observation that certain FOXP1 isoforms are capable of activating the NF-κB reporter in both B- and T-cell lines (Streubel et al. 2005; Barrans et al. 2004).
4.2.3.2 Translocation-Negative MALT Lymphoma and A20 Inactivation
While the analysis of chromosomal translocations has provided important insights into the pathogenesis of MALT lymphoma, it is important to note that the majority of MALT lymphomas – especially those of the ocular adnexa, salivary glands, and thyroid – do not harbor the aforementioned chromosome translocations (Du 2011). Work by Du and others has highlighted the role of A20 as a target of 6q23 deletion in translocation-negative MALT lymphomas (Du 2011; Chanudet et al. 2009). Through its dual ubiquitin-editing ability, the zinc finger protein A20 acts as a negative regulator of NF-κB activity attenuating the inflammatory and immune response (Rosebeck et al. 2011). Activation of immunoreceptors leads to rapid proteolytic cleavage and inactivation of A20 by MALT1 via its recruitment and formation of a complex with MALT1/BCL-10 (Rosebeck et al. 2011; Coornaert et al. 2008). Cleavage by MALT1 impairs the NF-κB inhibitory function of A20 (Malinverni et al. 2010). Additionally, A20 inhibits NF-κB signaling through disruption of the E2/E3 ubiquitin enzyme complex (Shembade et al. 2010). These observations have led some authors to suggest that restoring the molecular break via therapeutics targeting the proteolytic activity of MALT1 (rather than by inhibiting NF-κB activation directly) might represent a therapeutic alternative with lesser propensity for generalized immunodeficiency (Coornaert et al. 2008).
Du and others have stressed that neither the translocation product in translocation-positive MALT lymphoma nor A20 inactivation in translocation-negative MALT lymphoma is sufficient for malignant transformation. Rather, oncogenesis is thought to be dependent upon cooperation between genetic factors and immunologic drive. Evidence suggests that an important and complementary role is played by surface receptor stimulation in the translocation-positive setting and active immune response to antigen – including activation of NF-KB via TNF and other presently unidentified actors – in translocation-negative MALT lymphoma (Du 2011).
4.2.4 Waldenström’s Macroglobulinemia/Lymphoplasmacytic Lymphoma
Waldenström’s macroglobulinemia (WM) is a rare B-cell malignancy characterized by infiltration of the bone marrow with lymphoplasmacytic cells, hence the name lymphoplasmacytic lymphoma and the presence of an IgM monoclonal gammopathy in the serum (Owen et al. 2003). Accounting for 1–2 % of hematological malignancies, WM has an incidence of approximately three per million, with 1,400 new cases diagnosed each year in the United States (Fonseca and Hayman 2007). In contrast to the well-established clinical picture of the disease, less is known about its biological origins (Chng et al. 2006). The tumor clone is thought to evolve from a memory B cell that has undergone somatic hypermutation in the germinal center in the absence of antigenic selection and has failed to undergo clonotypic isotype class switching (Chng et al. 2006; Kriangkum and Taylor 2006; Sahota et al. 2002).
4.2.4.1 Cytokines and JAK/STAT Pathway Activation in the Tumor Microenvironment
The regulation of cell proliferation, dissemination, and trafficking through interactions between the malignant clone and the bone marrow microenvironment (BME) is a key area of research in WM (Ghobrial et al. 2011). Akin to multiple myeloma, a related plasma cell dyscrasia, the interaction with the BME results in cell proliferation and drug resistance through the activation of several proliferative signaling cascades including the PI3K, NF-κB, MAPK, and JAK/STAT pathways. BM stromal cells are crucial for the growth of WM cells, and expression of Jak1 and Stat3 protein is higher in the WM population than in controls (Hatjiharissi et al. 2007; Hodge and Ansell 2011). Aberrant activation of the JAK/STAT pathway is thought to depend on cytokine signaling with IL-6 likely playing a key role. IL-6 levels are increased in WM patients and decrease significantly during treatment (Hatzimichael et al. 2001). Elsawa and others have identified CCL5 as highly expressed in patients with WM (with CCL5 levels correlated to that of IL-6) and have established that CCL5 directly stimulates secretion of IL-6 in WM stromal cells through JAK/STAT signaling (Elsawa et al. 2011). Other additional cytokines, including IL-2, IL-7, IL-10, and IL-12, may also activate the JAK/STAT pathway and regulate IgM secretion (Hodge and Ansell 2011).
4.2.4.2 MYD88 and Oncogene Addiction
The TLR and IL-1 receptor adaptor protein MYD88 transduces signals to NF-κB transcription factors that induce expression of pro-survival and pro-proliferation cytokines (TNF, IL-6, IFNβ, IL-1β) and chemokines (CXCL1, CXCL2, CXCL10) (Ben-Neriah and Karin 2011). Via a complex interaction with its amino-terminal death domain, MYD88 acts to coordinate the IRAK-family kinases into a helical signaling complex (Lin et al. 2010). IRAK4 then phosphorylates IRAK1, enabling the recruitment of ubiquitin ligase TRAF6 and subsequent downstream signaling, including the NF-κB, p38 MAP kinase, and type I interferon pathways (Iwasaki and Medzhitov 2010; Staudt 2012). Recent work has implicated this innate immune signaling pathway in the pathogenesis of B-cell malignancies. In the case of activated B-cell-like subtype of diffuse large B-cell lymphoma (ABC-DLBCL), a somatic mutation in MYD88 giving rise to a mutant L265P variant (a single amino acid substitution located in the TIR domain) has been found to promote oncogenic activity via constitutive TLR signaling; this has been shown to occur via the assembly of the aforementioned complex leading to IRAK1 phosphorylation and downstream activation of the NF-κβ signaling pathway (Ngo et al. 2011). In WM, whole genome sequencing of bone marrow lymphoplasmacytic cells has documented expression of the same L265P somatic variant in a majority of cases (Treon et al. 2012). In this setting, greater phosphorylation of downstream proteins IRAK1, IκBα, NFκB-p65, and STAT3 was observed compared to wild type, and the disruption of MYD88 pathway signaling led the loss of constitutive activation of these proteins and induced apoptosis. (Treon et al. 2012; Yang et al. 2011) These findings, when combined with the observation from ABC-DLBCL that cell death after MYD88 knockdown may be rescued via mutant L265P but not via the wild type (Ngo et al. 2011), suggest that tumor maintenance depends upon the continued activity of MYD88, thus constituting a case of oncogene addiction (Weinstein 2002). Additionally, it is known that epigenetics also play a role in the regulation of cell proliferation within the bone marrow milieu, with recent studies highlighting the ability of miRNA-155 to modulate cellular growth (Sacco et al. 2011). WM cells are also driven, in part by miRNA-206, to an unbalanced expression of histone deacetylases (HDACs) and histone acetyl transferases (HATs) (Roccaro et al. 2010). Furthermore, both miRNA-155 and miRNA-21 have been shown to be overexpressed in WM cells compared to healthy donor B cells (Cao et al. 2011). MYD88 is also implicated in such microRNA dysregulation, with knockdown of MYD88 resulting in decreased levels of both miRNAs, as well as reduction in miRNA-21 following cell treatment with an IRAK 1/4 kinase inhibitor (Cao et al. 2011).
4.3 Molecular Pathogenesis of Peripheral T-Cell Lymphomas
Conversely to B-cell lymphomas, the molecular pathogenesis of peripheral T-cell lymphomas (PTCLs) has been the object of a limited number of studies. This certainly reflects the much lower incidence of the latter that corresponds to about 12 % of all lymphoid tumors all over the world (Swerdlow et al. 2008). Accordingly, there is a limited availability of fresh or frozen material for the application of novel high-throughput technologies that can provide substantial contributions in this setting. Nevertheless, there is the cogent need to deepen this issue, since the vast majority of PTCLs run a very aggressive clinical course with dismal prognosis (Vose et al. 2008). Such behavior may be at least in part due to the lack of sensitivity to anthracyclines as shown retrospectively by the International T-Cell Lymphoma Project (Vose et al. 2008) and experimentally in primary cell cultures by Piccaluga et al. (2007a).
In the following, attention will be paid to four varieties of PTCL that per se represent about two thirds of T-cell neoplasms, i.e., the not otherwise specified (NOS), angioimmunoblastic (AITL), and systemic ALK+ and ALK- anaplastic large-cell (ALCL) types (Jaffe et al. 2008). Notably, in the fourth edition of the WHO Classification of Tumours of Haematopoietic and Lymphoid Tissues, ALK-ALCL is quoted as a provisional entity – in contrast to the remaining ones that are regarded as distinct entities. Such attribution reflects the uncertainties as to the nosography of this tumor existing at the time of the classification writing, which have been largely overcome during the last 2 years (Agnelli et al. 2012; Piva et al. 2010). In spite of its “distinct” status, PTCL, NOS is by definition a Pandora’s box as diffuse large B-cell lymphoma (DLBCL) was in the past in the field of B-cell tumors. In fact, it includes all T-cell neoplasms that cannot be classified in one of the other categories.
In particular, two issues will be separately discussed: the histogenesis and pathogenetic mechanisms of these four types of PTCL.
4.3.1 Histogenesis
Two independent gene expression profiling (GEP) studies, simultaneously published by de Leval et al. (2007) and Piccaluga et al. (2007b), have provided important contributions concerning the histogenesis of AITL. First of all, they showed that AITL has a gene signature related to follicular helper T lymphocytes (FHT), i.e., the T-cell subset which takes part in the life and function of germinal center B cells. Such derivation found its phenotypic surrogate in the immunohistochemical detection of a series of markers (i.e., CD10, BCL6, PD-1, ICOS, SAP, C-MAF, CXCL13, and CCR5) that are physiologically expressed by FHT. Importantly, at least three of these antigens should be simultaneously detected to postulate the FHT origin of a given tumor (Laurent et al. 2010). In fact, one of them can incidentally occur in normal and pathological T-cell populations of variable derivation because of cell plasticity. Worthy of note is the fact that the postulated histogenesis of AITL explains some morphologic characteristics of the tumor (Dogan et al. 2008). Among these are the hyperplasia of follicular dendritic cells (FDCs) and the intimately intermingled B-cell component. The latter is usually EBV infected and represents the substrate for the development of an independent DLBCL that is encountered in 10–20 % of instances. The interaction between neoplastic T lymphocytes and B cells can also explain some of the clinical manifestations of AITL, such as polyclonal hypergammaglobulinemia, hemolytic anemia, and autoimmune phenomena in general.
Much more complex is the situation as to what PTCL, NOS is concerned. In 2007, Piccaluga et al. (2007a) first reported that by comparison with the gene signature of the main subsets of normal T-lymphocytes, the vast majority of PTCLs, NOS were closer to activated central memory T cells, only a small group revealing a cytotoxic profile. These findings were subsequently confirmed by Iqbal et al. (2010) who observed that cytotoxic tumors had an even worse prognosis than the others. Such assumption seems, however, to find an exception in the lymphoepithelioid variant of PTCL, NOS, also known as Lennert’s lymphoma, which behaves much better than all the tumors belonging to this category, in spite of its cytotoxic profile (Hartmann et al. 2011). Furthermore, de Leval et al. (2007) described a few PTCLs, NOS carrying an FHT-related signature, thus suggesting that the FHT derivation might not be exclusive of AITL. The latter assumption has found support in a series of immunohistochemical observations pertaining PTCL, NOS, with special reference to the follicular variant (Pileri et al. 2008). The latter may architecturally mimic follicular B-cell lymphoma, nodular lymphocyte-predominant Hodgkin lymphoma, or marginal zone B-cell lymphoma. Conversely to these conditions, the growth is however sustained by clear cells similar to the ones seen in AITL. In the last edition of the WHO Classification (Pileri et al. 2008), such variant was maintained distinct from AITL for the following reasons: (a) the occurrence of a specific translocation [t(5;9)(q33;q22) fusing ITK to SYK] in about 25 % of cases (Streubel et al. 2006), (b) the limited extent of the disease at the time of presentation, (c) the lack of the symptomatic complex of AITL, which however has been recently questioned (Miyoshi et al. 2012), and (d) the absence of high endothelial venules (HEV) and FDC hyperplasia. In the meanwhile, the usage of markers raised against FHT-related antigens has shown that, besides the follicular variant, other PTCLs, NOS growing diffusely and consisting of clear cells but lacking the hallmark of AITL (i.e., HEV and FDC hyperplasia) are also related to FHT (Agostinelli et al. 2011). This prompts the question whether or not a new category of T-cell tumors should be envisaged ranging from AITL to cases belonging to the PTCL, NOS morphologic spectrum, all provided with the same derivation. Prospective clinical-pathological studies are warranted to definitely assess this point.
ALCLs, both ALK+ and ALK-, stem from activated cytotoxic T lymphocytes that have partially lost the expression of T-cell-associated antigens (Piccaluga et al. 2007a; Piva et al. 2010). They are morphologically and phenotypically undistinguishable with only one exception the lack of the small-cell variant in the setting of ALK- ALCL. Clinically, ALK- ALCL runs a more aggressive clinical course than the ALK+ one (Delsol et al. 2008; Mason et al. 2008). Retrospective studies have suggested that ALK- ALCL has anyhow a better prognosis than PTCL, NOS with CD30 expression (Savage et al. 2008), thus supporting the decision taken at the time of the WHO Classification writing to maintain it as a provisional entity. The assignment of a given tumor with the typical morpho-phenotypic features of ALCL (i.e., presence of hallmark cells, intrasinusoidal diffusion, cohesive growth pattern, strong CD30 expression, EMA positivity, and activated cytotoxic profile) to one or the other of the two categories is based on the occurrence of a translocation [more often t(2;5)(p23;q35)] leading part of the transcriptional domain of ALK under the control of a partner gene (usually NPM) (Delsol et al. 2008; Barreca et al. 2011; Inghirami and Pileri 2011). The newly formed hybrid gene encodes for a chimeric protein that can be easily detected with antibodies raised against the ALK protein. GEP studies have largely contributed to clarify the relationships between ALK+ and ALK- ALCLs and the borders between ALK- ALCL and PTCL, NOS, more than to assess their histogenesis. Thompson et al. (2005) were the first to apply this approach demonstrating the feasibility of this analysis in distinguishing ALK+ from ALK- ALCL, although both entities were found to share a common profile, suggesting putative common pathogenetic lesions. These findings were subsequently confirmed by Lamant et al. (2007) who demonstrated that a limited set of genes, including BCL6, C/EBPb, SERPINA1, and PTPN12, were preferentially expressed by ALK+ ALCL compared with ALK- ALCL. Moreover, common type ALCL and morphologic variants (small-cell, lympho-histiocytic and “mixed” variants) could also be distinguished (Lamant et al. 2007). Later on, Piva et al. (2010) demonstrated that ALCL could be differentiated from PTCL, NOS by a relatively small classifier and that ALK+ and ALK- cases could be stratified by the expression of selected genes. These included perforin, IL2RA, and GAS1. More recently, Agnelli et al. (2012) have undertaken a transcriptional profiling meta-analysis of 309 cases, including ALCL and other primary T-NHL samples. Pathway discovery and prediction analyses defined a minimum set of genes capable to recognize ALK- ALCL. Application of RT-qPCR in independent data sets from cryopreserved and formalin-fixed paraffin-embedded (FFPE) samples validated a three-gene model (TNFRSF8, BATF3, TMOD1) able to successfully separate ALK- ALCL from PTCL-NOS, with overall accuracy near 97 %.
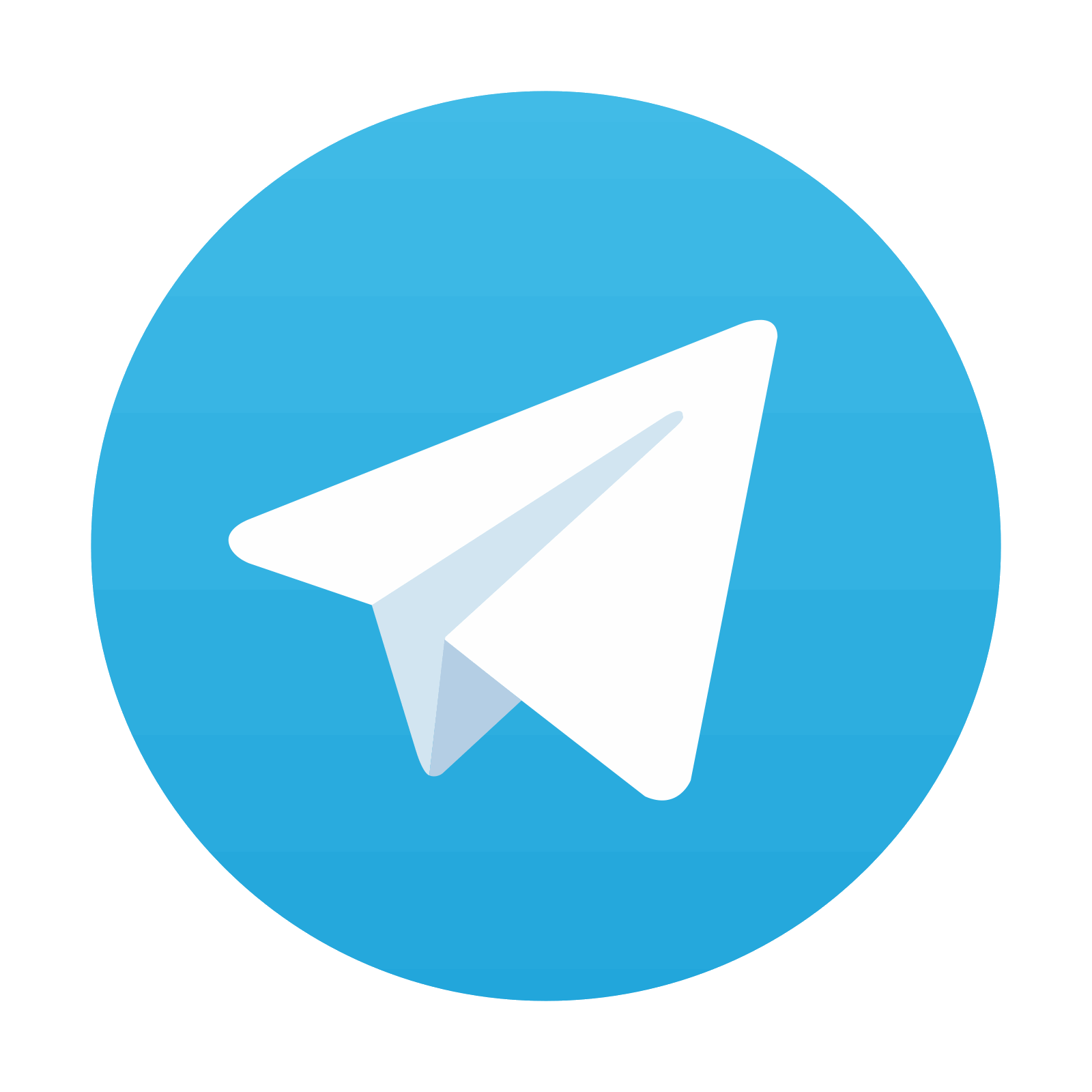
Stay updated, free articles. Join our Telegram channel
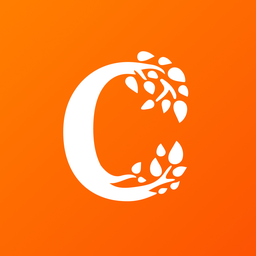
Full access? Get Clinical Tree
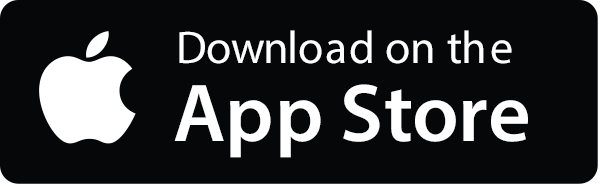
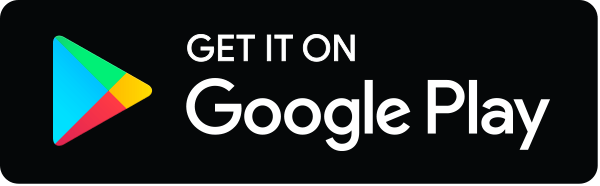