INTRODUCTION
SUMMARY
Iron is a component of nearly all living organisms. It plays an important metabolic role, particularly in electron transfer reactions. Most of the iron in the human body is incorporated into the hemoglobin of circulating red cells, which contain approximately 1 mg of iron per 1 mL of packed cells. Smaller amounts of iron are present in myoglobin and in many enzymes. Iron is stored within cells inside ferritin and circulates in plasma bound to transferrin. Because little iron is lost from the body under normal circumstances, the iron content of the body is controlled by modulating dietary iron absorption. Iron absorption increases in the presence of iron deficiency and it decreases when there is iron overload. The absorption of inorganic iron involves a ferrireductase and a divalent iron transporter, DMT-1, on the gastrointestinal luminal apical membranes of enterocytes, and ferroportin and hephaestin, located on the basolateral enterocyte membranes, in contact with blood. In contrast to elemental iron, heme iron is absorbed by a distinct pathway, which is still not well understood.
Systemic iron homeostasis is orchestrated by the hepatic peptide hormone hepcidin, which regulates plasma iron concentrations, the absorption of dietary iron, and the release of iron from macrophages involved in iron recycling and storage and from hepatocytes that store iron. The cellular iron exporter ferroportin serves as the receptor for hepcidin and is destroyed when the complex is formed. This impairs transport from intestinal mucosal cells, from macrophages and from hepatocytes into the plasma, and lowers iron absorption and release from stores. Hepcidin decreases plasma iron levels by causing iron to be sequestered within cells, predominantly in macrophages or enterocytes, the latter of which are then shed along with their absorbed iron. Once ferric iron enters the plasma, it is bound by transferrin, which, after forming a complex with the transferrin receptor, transports the metal into cells. The transferrin receptor is internalized together with bound transferrin and iron, and the iron is released inside the cell into an acidified vacuole. The transferrin receptor then recycles to the cell surface.
Cellular iron homeostasis is largely achieved through posttranscriptional regulation of key proteins involved in iron transport, storage and utilization. The synthesis of these proteins is regulated by binding of one of the iron-regulatory proteins (IRPs) to iron-responsive elements (IREs) located within stem loop structures of the corresponding messenger ribonucleic acids (mRNAs). IRP-1 is cytoplasmic aconitase that binds to the IRE when it is not complexed with iron and does not bind when iron is present; IRP-2, a closely related protein, is destabilized by the presence of iron. When IRPs bind to IREs at the 5′ end of the mRNA, they prevent translation; when they bind at the 3′ end, they stabilize the mRNA.
Iron is a key element in the metabolism of nearly all living organisms. Iron is a component of heme, which is the active site of electron transport in cytochromes and cytochrome oxidase involved in mitochondrial energy generation. The heme moiety of hemoglobin and myoglobin binds O2, providing the means to transfer O2 from the lungs to tissues and to store it. Heme is also the active site of peroxidases that protect cells from oxidative injury by reducing peroxides to water or generate microbicidal hypochlorite in granulocytes. DNA synthesis requires the enzyme ribonucleotide reductase to convert ribonucleotides to deoxyribonucleotides. Neither bacteria nor nucleated cells proliferate when the supply of iron is insufficient.
Acronyms and Abbreviations:
ABCB10, ATP-binding cassette (ABC) transporter in the inner membrane of mitochondria; ALA synthase, aminolevulinic acid synthase; BMP, bone morphogenetic protein; dcytb, duodenal cytochrome b; DMT, divalent metal transporter; GDF15, growth differentiation factor 15; HFE, human hemochromatosis protein; HRG1, heme transporter; IL, interleukin; IRE, iron-responsive element; IRP, iron-regulatory protein; NADPH, nicotinamide adenine dinucleotide phosphate; Nramp1, natural resistance-associated macrophage protein one; STEAP3, six-transmembrane epithelial antigen of prostate 3; TfR, transferrin receptor.
In the previous edition, this chapter was written by Ernest Beutler and portions of that chapter have been retained.
DISTRIBUTION OF IRON IN THE AVERAGE PERSON
Table 42–1 summarizes the most important iron compartments.
Hemoglobin, which is 0.34 percent iron by weight, contains approximately 2 g of body iron in men and 1.5 g in women. One mL of packed erythrocytes contains approximately 1 mg of iron. Because the life span of human erythrocytes is approximately 120 days, every day 1/120 of the iron in hemoglobin is recycled by macrophages and returned to the plasma, from where it is largely delivered to marrow erythroblasts for incorporation into newly synthesized hemoglobin.
Iron is stored either as ferritin or as hemosiderin. The former is water-soluble; the latter is water-insoluble. The protein ferritin is composed of 24 similar or identical subunits arranged as 12 dimers forming a dodecahedron that approximates a hollow sphere with a cavity capable of storing up to 4500 Fe atoms as hydrous ferric oxide polymers.1,2 The ferritin subunits are of H (heavy) or L (light) type. H subunits have ferroxidase activity, thereby enabling ferritin to take up or release iron quite rapidly. Ferritin that is rich in H subunits takes up iron more readily, but retains it less avidly than does ferritin composed predominantly of L subunits. Much of the storage iron in liver and spleen is in ferritin containing mostly L subunits.
Ferritin is found in virtually all cells of the body and also in tissue fluids. In plasma ferritin is present in minute concentrations. It is glycosylated and largely composed of L subunits. Except under conditions of inflammation, the plasma (serum) ferritin concentration usually correlates with total-body iron stores, making measurement of serum ferritin levels important in the diagnosis of disorders of iron metabolism.
The size of the iron storage compartment is quite variable. Normally, in adult men, it amounts to 800 to 2000 mg; in adult women, it is a few hundred milligrams. The mobilization of storage iron from ferritin involves the reduction of Fe3+ to Fe2+, its release from the core crystal and its diffusion out of the apoferritin shell. As it passes from cytosol to plasma, it must be reoxidized to Fe3+, either by hephaestin or ceruloplasmin in the cell membrane or by ceruloplasmin in plasma, before it binds to transferrin. Alternatively, iron may be released from ferritin by autophagy followed by lysosomal degradation.3
Hemosiderin is found predominantly in macrophages. Microscopically, in unstained tissue sections or marrow films it appears as clumps or granules of golden refractile pigment. Under pathologic conditions, it may accumulate in large quantities in almost every tissue of the body. Hemosiderin is chemically similar to the iron core of ferritin and may be derived from ferritins whose protein shells have been digested in lysosomes.
Myoglobin is structurally similar to hemoglobin, but it is monomeric rather than tetrameric: Each myoglobin molecule consists of a heme group nearly surrounded by polypeptide loops of the 154 amino acid protein. It is present in small amounts in all skeletal and cardiac muscle cells, where it may serve as an oxygen reservoir to protect against cellular injury during periods of oxygen deprivation and may scavenge nitric oxide and reactive oxygen species.4
The existence of a cellular labile iron pool was postulated from studies of the rate of clearance of injected 59Fe from plasma.5 Iron leaves the plasma and enters the interstitial and intracellular fluid compartments for a brief time before it is incorporated into heme or storage compounds. Some of the iron reenters plasma, causing a biphasic curve of 59Fe clearance 1 to 2 days after injection. The change in slope defines the size of the labile pool, normally 80 to 90 mg of iron. It is now sometimes considered to be equivalent to the chelatable iron pool.6
Tissue iron (exclusive of hemoglobin, ferritin, hemosiderin, myoglobin, and the labile compartment) normally amounts to 6 to 8 mg. This includes cytochromes and other iron-containing enzymes. Although a small compartment, it is an extremely vital one and is sensitive to iron deficiency.7,8
From the standpoint of its total iron content, normally about 3 mg, the transport compartment of plasma is the smallest but the most active of the iron compartments: Its iron, almost entirely carried by transferrin, normally turns over at least 10 times each day. This is the common pathway for interchange of iron between compartments.
Transferrin is a dumbbell-shaped glycoprotein with a Mr of approximately 80 kDa where each of the two globular domains contains a binding cleft for Fe3+.9,10,11 Normally, approximately one-third of the transferrin iron-binding sites are occupied by iron. Human plasma normally contains approximately 25 to 45 μM (200 to 360 mg/dL) transferrin, capable of binding 50 to 90 μM iron but carrying only 10 to 30 μM (50 to 180 mcg/dL) iron. Apotransferrin (transferrin devoid of iron) is synthesized by hepatocytes and by cells of the monocyte–macrophage system.12,13
DIETARY IRON
Average American adult men and women ingest 9 to 10 mg and 12 to 14 mg of iron daily, respectively.14 The amount of iron absorbed by a normal adult male need only balance the small amount that is excreted, mostly in the stool, approximately 1 mg/day.15 More iron is needed during growth periods or after blood loss. In women, iron absorbed must be sufficient to replace that lost through menstruation or diverted to the fetus or milk during and after pregnancy. Table 42–2 shows the age- and gender-specific recommended dietary allowances for iron.16
Age | Male | Female | Pregnancy | Lactation |
---|---|---|---|---|
Birth to 6 months | 0.27 mg* | 0.27 mg* | ||
7–12 months | 11 mg | 11 mg | ||
1–3 years | 7 mg | 7 mg | ||
4–8 years | 10 mg | 10 mg | ||
9–13 years | 8 mg | 8 mg | ||
14–18 years | 11 mg | 15 mg | 27 mg | 10 mg |
19–50 years | 8 mg | 18 mg | 27 mg | 9 mg |
51+ years | 8 mg | 8 mg |
In meat-eaters in Western countries, heme from hemoglobin and myoglobin normally comprises approximately 15 percent of dietary iron but is much more efficiently absorbed than nonheme iron, and promotes the absorption of nonheme iron.17 The absorption of nonheme dietary iron is strongly affected by iron-binding components of food. Oxalates, phytates, and phosphates complex with iron and retard its absorption, whereas simple reducing substances, such as hydroquinone, ascorbate, lactate, pyruvate, succinate, fructose, cysteine, and sorbitol, increase iron absorption.18 Iron-fortified cereals are major sources of iron in countries where fortification is practiced, but cooking in iron pots may also provide important exogenous iron.17 Gastric acid secretion, the transit time, and mucus secretion all play roles in iron absorption. Red wine, contrary to popular belief, inhibits iron absorption, probably because of the presence of polyphenols.19 In mice, alcohol suppresses the response of hepcidin to iron,20 and this may contribute to iron loading that is seen in some alcoholic subjects.
IRON ABSORPTION
Iron normally enters the body through the gastrointestinal tract, mostly through the enterocytes of the duodenum. The amount of iron absorbed is normally tightly regulated according to body needs. Active erythropoiesis and/or iron deficiency increase absorption; iron overload and systemic inflammation decrease absorption. Nevertheless, the amount of iron absorbed increases with the administered dose even though the percentage absorbed decreases (Fig. 42-1). Accidental or deliberate ingestion of large doses of medicinal iron can therefore cause iron intoxication.
Figure 42–1.
The relationship between oral iron dosage and amount of iron absorbed in humans. When the logarithm of the dose is plotted against the logarithm of the amount of iron absorbed, a rectilinear relationship is observed. Thus, at all levels, the greater the dose of iron, the more is absorbed, although the percent of the dose that is absorbed progressively declines. (Reproduced with permission from Mackenzie B, Garrick MD: Iron Imports. II. Iron uptake at the apical membrane in the intestine. Am J Physiol Gastrointest Liver Physiol 289(6):G981–G986, 2005.)
Understanding the mechanism of iron absorption has been made more difficult by the fact that the pathways for the uptake of inorganic iron and of heme by enterocytes are different but seem to merge within the intestinal cell where heme is converted to inorganic iron. How much heme (if any) is exported intact by enterocytes and bound by plasma heme-binding protein hemopexin is not clear, but hemopexin knockout mice show minor retention of iron in duodenal enterocytes without any effect on systemic iron homeostasis,21 arguing against a major contribution from this mechanism, at least in mice. Efforts to identify the apical heme import mechanism in enterocytes have not yet been definitive.22
Following the reduction of ferric iron to ferrous iron, in part by duodenal cytochrome b (dcytb) reductase,23 ferrous iron is transported into the intestinal villus cell by the divalent metal transporter (DMT)-1.24,25 How iron transits within the enterocytes is not yet known. Basolateral export of ferrous iron is mediated by ferroportin26,27,28 in association with hephaestin29 and plasma ceruloplasmin30 to oxidize iron to the ferric state. Ferric iron is taken up by plasma apotransferrin. Figure 42–2 illustrates some of the steps that are thought to regulate iron transport across the mucosal cell.
Figure 42–2.
Schematic of iron uptake from the intestine and transfer to the plasma by an intestinal villus cell. Nonheme dietary iron includes Fe(II) and Fe(III) salts and organic complexes. Fe3+ is reduced to Fe2+ by ascorbic acid and apical membrane ferrireductases that include duodenal cytochrome b (dcytb). The acid microclimate at the brush-border provides an H+ electrochemical potential gradient to drive transport of Fe2+ via the divalent metal-ion transporter (DMT-1) into the enterocyte. DMT-1 may also contribute to the absorption of other nutritionally important metal ions (e.g., Mn2+). Heme can be taken up by endocytosis, and Fe2+ is liberated within the endosome/lysosome, but the molecular identity of proteins involved, including heme carrier protein 1 (HCP1), is yet to be elucidated. Basolateral export of Fe2 may be mediated by ferroportin in association with hephaestin. Fe2Tf, diferric transferrin; HO, heme oxygenase. (Data from Smith MD, Pannacciulli IM: Absorption of inorganic iron from graded doses: its significance in relation to iron absorption tests and mucosal block theory. Br J Haematol 4(4):428–434, 1958.)
In humans, the destruction and production of erythrocytes generates most of the iron flux in and out of plasma (20 to 25 mg/day recycled in adults compared to 1 to 2 mg/day absorbed). Iron from other cell types is likely also recycled, but this source contributes little to iron flux and has not been studied. Destruction of aged erythrocytes and hemoglobin degradation occur within macrophages (Chap. 32). This proceeds at a rate sufficient to release approximately 20 percent of the hemoglobin iron from the cell to the plasma compartment within a few hours. Approximately 80 percent of this iron is rapidly reincorporated into hemoglobin. Thus, 20 to 70 percent of the hemoglobin iron of nonviable erythrocytes reappears in circulating red cells in 12 days. The remainder of the iron enters the storage pool as ferritin or hemosiderin and then turns over very slowly. In normal subjects, approximately 40 percent of this iron remains in storage after 140 days. When there is an increased iron demand for hemoglobin synthesis, however, storage iron may be mobilized more rapidly.31 Conversely, in the presence of infection or another inflammatory process (e.g., ulcerative colitis or malignancy), iron is more slowly reused in hemoglobin synthesis and is associated with anemia (Chap. 37).32,33
As human erythrocytes age during their average 120-day life span, they shrink, stiffen, and their membranes accumulate markers of senescence.34 These changes eventually trigger phagocytosis by splenic or hepatic sinusoidal macrophages. Macrophages also take up the products of intravascular hemolysis, including hemoglobin (bound by haptoglobin) and heme (bound by hemopexin), using specific endocytic receptors for the complexes.35 The vesicles involved in phagocytosis and endocytosis must fuse with lysosomes to digest cellular materials or protein complexes and to free heme from hemoglobin. The membrane complex of nicotinamide adenine dinucleotide phosphate (NADPH) cytochrome c reductase, heme oxygenase 1, and biliverdin reductase releases ferrous iron from heme and simultaneously protects erythrophagocytosing macrophages from heme-induced toxicity.36 The subcellular location of the conversion of heme to iron is not known with certainty. Heme oxygenase 1 is mostly located in the endoplasmic reticulum in erythrophagocytic macrophages37 with the catalytic face in the cytosol, and little, if any, heme oxygenase in the phagosomal membrane. Moreover, the phagosomal membrane is enriched in the heme transporter HRG1,38 and macrophage heme has a signaling role in inducing various proteins involved in macrophage iron metabolism, indicating that it may leave the phagosome, and the heme oxygenase-1–mediated release of iron may occur in the cytoplasm. However, the ferrous iron transporter Nramp1, and perhaps DMT-1, may also participate in subcellular iron transport.39 Ultimately, depending on systemic iron requirements, the released ferrous iron is either exported to plasma via ferroportin40 or trapped in macrophage cytoplasmic ferritin. By a mechanism potentially important at the low oxygen tensions found in some tissues, plasma ceruloplasmin41,42,43 catalyzes the conversion of ferrous to ferric iron, the form of iron loaded to plasma transferrin for systemic distribution.
The mechanism by which body iron content is regulated by the modulation of iron absorption has been a subject of intense interest for the past 65 years. It has now become clear that intestinal iron absorption, plasma iron concentrations, and tissue distribution of iron are subject to endocrine regulation similar to that of other simple nutrients, for example, glucose or calcium, albeit in a somewhat more complex fashion.
Hepcidin, a 25-amino-acid peptide hormone with 4 disulfide bonds,44,45,46,47 is produced predominantly by hepatocytes and plays a central role in systemic iron homeostasis. Hepcidin regulates plasma iron concentrations by controlling the absorption of iron by the intestinal epithelial enterocytes and its release from iron-recycling macrophages and hepatocytes involved in iron storage. The structural similarity of hepcidin and a class of antimicrobial peptides termed defensins suggests that the hormone evolved from the latter to modulate iron homeostasis as a mechanism of body defense against microorganisms. Overexpression of hepcidin results in marked iron-deficiency anemia in mice48 and a refractory anemia resembling the anemia of chronic inflammation in humans,49
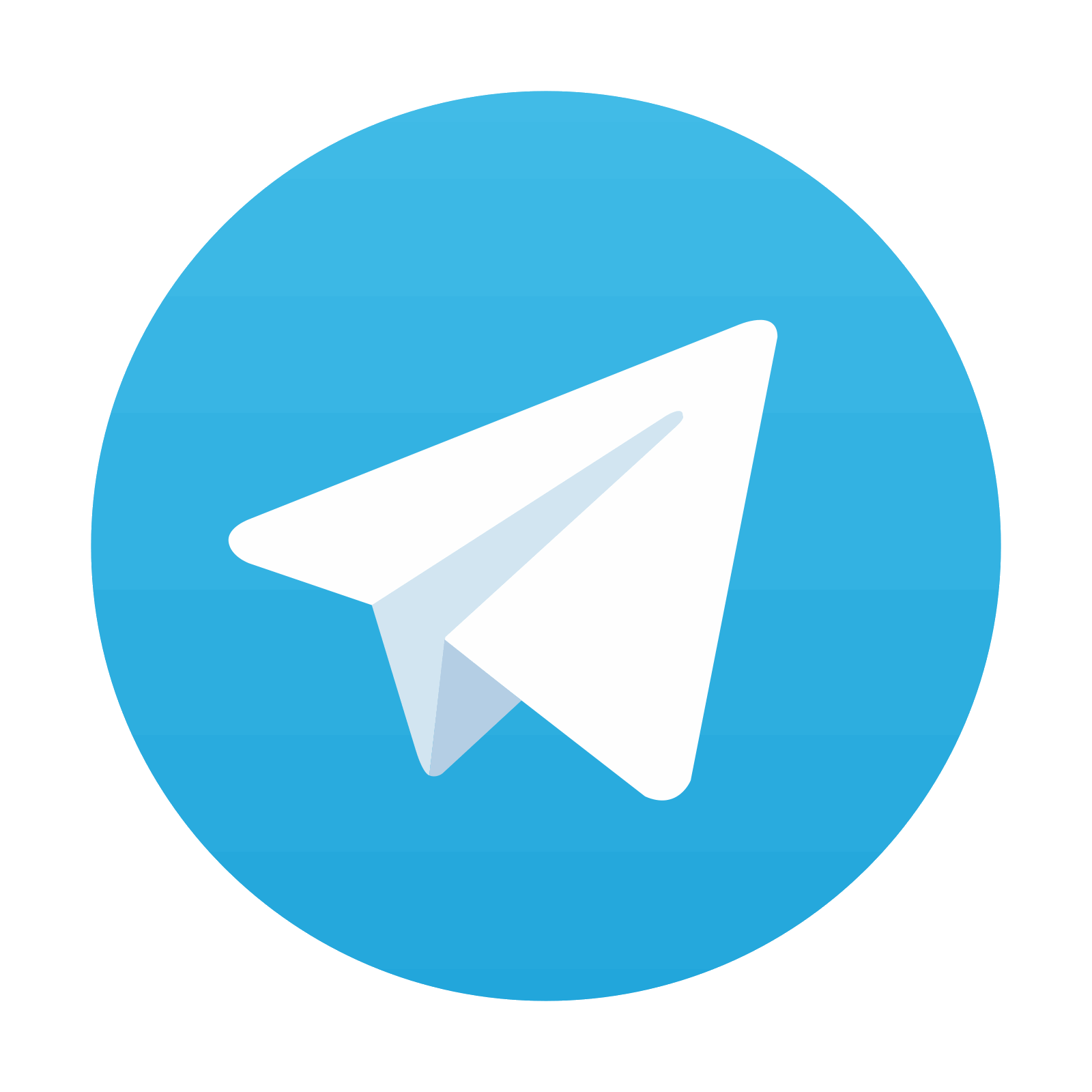
Stay updated, free articles. Join our Telegram channel
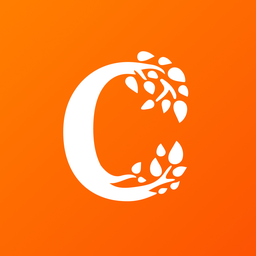
Full access? Get Clinical Tree
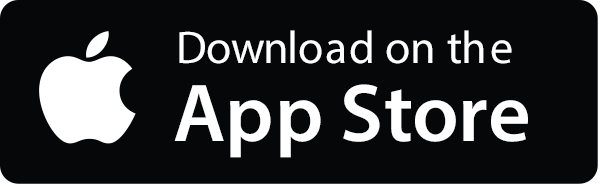
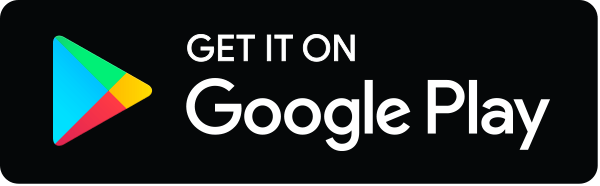
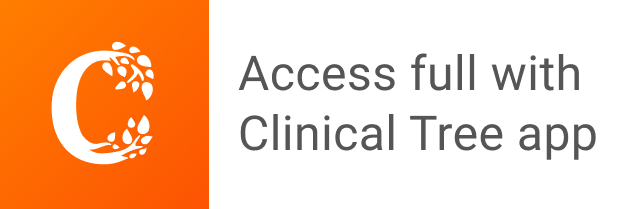