INTRODUCTION
SUMMARY
During embryogenesis, hematopoiesis occurs in spatially and temporally distinct sites, including the extraembryonic yolk sac, the fetal liver, and the preterm marrow. The development of primitive erythroblasts in the yolk sac is critical for embryonic survival. Primitive erythroblasts differentiate within the vascular network rather than in the extravascular space and circulate as nucleated cells. Although it is widely assumed that primitive red cells remain nucleated throughout their life span, primitive erythroblasts ultimately enucleate upon terminal differentiation. After 7 weeks of gestation, hematopoietic progenitors are no longer detected in the yolk sac. Hematopoietic stem cells emerge from major arterial vessels at 5 weeks of gestation. The liver serves as the primary source of red cells from the 9th to the 24th week of gestation. Like primitive erythropoiesis in the yolk sac, definitive erythropoiesis in the fetal liver is necessary for continued survival of the embryo. In contrast to the yolk sac, where hematopoiesis is restricted to maturing primitive erythroid, macrophage, and megakaryocytic cells, hematopoiesis in the fetal liver consists of definitive erythroid, megakaryocyte, and multiple myeloid, as well as lymphoid lineages. Hematopoietic cells are first seen in the marrow of the 10- to 11-week embryo, and they remain confined to the diaphyseal regions of long bones until 15 weeks of gestation. Lymphopoiesis is present in the lymph plexuses and the thymus beginning at 9 weeks of gestation. Yolk sac stem cells were first thought to seed the liver and eventually the marrow. However, later experiments in avian and amphibian embryos indicate that the hematopoietic stem cells that seed the marrow arise within the body of the embryo proper rather than from the yolk sac. The aorta-gonad-mesonephros (AGM) region generates hematopoietic stem cells that seed the liver and the marrow to provide lifelong hematopoiesis. Hemoglobin (Hgb) Gower-1 (ζ2ε2) is the major hemoglobin in embryos younger than 5 weeks. Hgb F (α2γ2) is the major hemoglobin of fetal life. The fetal hemoglobin concentration in blood decreases after birth by approximately 3 percent per week and is generally less than 2 to 3 percent of the total hemoglobin by 6 months of age. The mean hemoglobin level in cord blood at term is 16.8 g/dL, with 95 percent of the values falling between 13.7 and 20.1 g/dL. The red cells of the newborn are macrocytic, with a mean cell volume in excess of 110 fL/cell. The red cell, hemoglobin, and hematocrit values decrease only slightly during the first week after birth, but decline more rapidly in the following 5 to 8 weeks, producing the physiologic anemia of the newborn. The absolute number of neutrophils in the blood of term and premature infants is usually greater than that found in older children. Segmented neutrophils are the predominant leukocytes in the first few days after birth. As their number decreases, the lymphocyte becomes the most numerous cell type and remains so during the first 4 years of life. Phagocytosis of bacteria by neutrophils from premature and term infants is normal. Bactericidal activity varies according to the conditions of testing and the clinical status of the neonates. The platelet counts in term and preterm infants are between 150 and 400 × 109/L, comparable to adult values. The absolute number of lymphocytes in the newborn is equivalent to that in older children, with lower values in premature infants at birth. The absolute number of CD3+ and CD4+ (helper/inducer phenotype) T-cell subsets in blood of newborns is significantly higher than in adults. Humoral (B-cell) immunity also develops early in gestation, but it is not fully active until after birth. In the newborn, approximately 15 percent of lymphocytes have immunoglobulin on their surface, with all immunoglobulin isotypes represented. The term newborn has reduced mean plasma levels (<60 percent of adult levels) of factors II, IX, X, XI, and XII, prekallikrein, and high-molecular-weight kininogen. In contrast, the plasma concentration of factor VIII is similar and von Willebrand factor is increased compared to older children and adults.
Acronyms and Abbreviations:
ADP, adenosine diphosphate; AGM, aorta-gonad-mesonephros; ATP, adenosine triphosphate; ATPase, adenosine triphosphatase; BFU-E, burst-forming unit–erythroid; BPG, bisphosphoglycerate; BPI, bacterial permeability-increasing protein; cAMP, cyclic adenosine monophosphate; CFU-E, colony-forming unit–erythroid; CFU-GEMM, colony-forming unit–granulocyte-erythroid-monocyte-macrophage; CFU-GM, colony-forming unit–granulocyte-monocyte; CFU-Meg, colony-forming unit–megakaryocyte; G-CSF, granulocyte colony-stimulating factor; GM-CSF, granulocyte-monocyte colony-stimulating factor; IL, interleukin; MCV, mean cell volume; NBT, nitroblue tetrazolium; NK, natural killer; RDW, red cell distribution width; SIDS, sudden infant death syndrome; TNF, tumor necrosis factor; TPO, thrombopoietin.
FETAL HEMATOLYMPHOPOIESIS
During embryogenesis, hematopoiesis occurs in spatially and temporally distinct sites, including the extraembryonic yolk sac, the fetal liver, the thymus, and the preterm marrow. The origin of hematopoietic cells is closely tied to gastrulation, the formation of mesoderm cells, and to the emergence of the endothelial lineage. Hematopoiesis is first established soon after implantation of the blastocyst, with the appearance of primitive erythroid cells in blood islands of the yolk sac beginning at day 18 of gestation.1 The spatial and temporal association of embryonic red cells and endothelial cells in these blood islands suggests that the transient erythromyeloid potential of the yolk sac arises from hemangioblast precursors that also contain endothelial potential.2 This concept is supported by in vitro studies of human embryonic stem cells cultured as embryoid bodies.3,4 Hematopoietic stem cells, containing erythromyeloid and lymphoid potential, subsequently arise from intraembryonic vasculature, particularly the aorta (Fig. 7–1). These hematopoietic stem cells provide for fetal and long-term postnatal blood cell production. The ontogeny of the hematopoietic system remains a topic of active research using mammalian and several nonmammalian model systems.
Figure 7–1.
Hypothetical model of human hematopoietic ontogeny based on amphibian, avian, murine, and human developmental data. The yolk sac provides two transient populations of committed progenitors that are thought to arise from a mesoderm-derived hemangioblast (HB) precursor. The first wave of progenitors produces primitive erythroblasts (PRIM. ERY.) (see text). The second wave produces burst-forming unit–erythroid (BFU-E) and several myeloid progenitors (MP) that seed the liver. Long-term hematopoietic stem cells (HSCs) arise later in the aorta-gonad-mesonephros (AGM) region that subsequently populate the liver and ultimately the marrow to generate the full panoply of definitive hematopoiesis. The HSCs from liver also provide naïve lymphoid cells to the thymus, and T-lineage maturation occurs there.
“Primitive” red cells derived from the yolk sac constitute a distinct transient erythroid lineage that differs from “definitive” red cells that subsequently mature in the fetal liver and marrow. The development of primitive erythroblasts is critical for embryonic survival. In the mouse, targeted disruption of the transcription factors SCL (TAL1), LM02 (RBTN2), and GATA-1 abrogates primitive erythropoiesis in the yolk sac and leads to early embryonic death.5,6,7 In the human, primitive erythroblasts begin to enter the embryo proper at days 21 to 22 of gestation with the onset of cardiac contractions8 and circulate until approximately 12 weeks of gestation. Yolk sac erythroblasts have several characteristics that distinguish them from their later definitive counterparts. Primitive erythroblasts circulate as nucleated cells, accumulating embryonic hemoglobins and completing terminal differentiation within the vascular network.9 Because of their extremely large size, with an estimated MCV of >400 fL/cell, yolk sac erythroblasts have been termed megaloblasts. Although it is widely assumed that primitive red cells remain nucleated throughout their life span, human primitive erythroblasts, like their murine counterparts, ultimately enucleate upon terminal differentiation.10,11,12
In the mouse, primitive red cells are derived from a transient population of primitive erythroid progenitors that is confined to the yolk sac.13 Ultrastructural examination of the human yolk sac reveals the presence, not only of primitive erythroblasts, but also of macrophage cells and megakaryocytes.11 These findings are consistent with hematopoietic progenitor studies in the mouse embryo and in human embryonic stem cells differentiated in vitro, which support the concept that “primitive” hematopoiesis in the yolk sac consists of primitive erythroid, macrophage, and megakaryocyte lineages.13,14,15
The initial wave of primitive erythroid progenitors is followed by a second wave of yolk sac–derived definitive erythroid progenitors, termed burst forming units–erythroid (BFU-E). BFU-E are present in the human yolk sac as early as 4 weeks’ gestation and are found in the fetal liver by 5 weeks’ gestation.16 These findings suggest that the fetal liver is initially seeded by hematopoietic progenitors derived from the yolk sac (see Fig. 7–1).17 Erythroid and nonerythroid progenitors are evident also in the nonliver regions of the embryo proper.18 After 7 weeks’ gestation, hematopoietic progenitors are no longer detected in the yolk sac.19
The liver serves as the primary source of red cells from the 9th to the 24th weeks of gestation. Between 7 and 15 weeks’ gestation, 60 percent of the liver cells are hematopoietic.20 Erythroid cells differentiate in close physical association with macrophages and extrude their nuclei prior to entering the blood. These fetal-liver–derived definitive “macrocytes” are smaller than yolk sac–derived primitive megaloblasts and contain one-third the amount of hemoglobin. Differentiation of murine erythroid cells in the fetal liver is critically dependent on erythropoietin signaling through its receptor and the Janus kinase 2 (JAK2) pathway.21,22 Fetal-liver–derived erythroid progenitors can differentiate in vitro with erythropoietin alone, in contrast to adult marrow-derived BFU-E, which requires erythropoietin plus interleukin (IL)-3 or stem cell factor.23,24 Erythropoietin transcripts are present during the first trimester in the liver, which remains a primary site of erythropoietin transcription throughout fetal life.25 Erythropoietin transcripts also are present in the developing human kidney as early as 17 weeks’ gestation and increase after 30 weeks.25 Like primitive erythropoiesis in the yolk sac, definitive erythropoiesis in the fetal liver is necessary for continued survival of the embryo. Targeted disruption of the c-myb transcription factor in the mouse blocks fetal liver erythropoiesis and leads to fetal death.26 This mutation does not affect primitive erythropoiesis, indicating fundamental differences in the transcriptional regulation of these distinct forms of erythropoiesis.
In contrast to the yolk sac, where hematopoiesis is restricted primarily to erythromyeloid lineages, hematopoiesis in the fetal liver eventually will consist of definitive erythroid, megakaryocyte, multiple myeloid, as well as, lymphoid lineages. Megakaryocytes are present in the liver by 6 weeks’ gestation. Platelets are first evident in the circulation at 8 to 9 weeks’ gestation.20 Granulopoiesis is present in the liver parenchyma as early as 7 weeks’ gestation and small numbers of circulating leukocytes are present at the 11th week of gestation. Despite the low number and immature appearance of hepatic neutrophils, the fetal liver contains abundant hematopoietic progenitor cells, including the multipotential colony-forming unit–granulocyte-erythroid-monocyte-macrophage (CFU-GEMM) and colony-forming unit–granulocyte-monocyte (CFU-GM).27 CFU-GM growth depends upon several cytokines, including granulocyte colony-stimulating factor (G-CSF), granulocyte-monocyte colony-stimulating factor (GM-CSF), and interleukins. When compared to adult marrow-derived myeloid progenitors, these fetal liver-derived myeloid progenitors have a similar dose–response in vitro to G-CSF.28 G-CSF is expressed by hepatocytes at 14 weeks’ gestation.29
Lymphopoiesis is present in the lymph plexuses and the thymus beginning at 9 weeks’ gestation.20 B cells with surface immunoglobulin (Ig) M are present in the liver, and circulating lymphocytes also are seen at 9 weeks’ gestation. T lymphocytes are found only rarely before 12 weeks’ gestation.30 Lymphocyte subpopulations are detected by 13 weeks’ gestation in fetal liver.31 Absolute numbers of major lymphoid subsets in 20- to 26-week-old fetuses, as defined by the antigens CD2, CD3, CD4, CD8, CD16, CD19, and CD20, are similar to those in newborns (see “Neonatal Lymphopoiesis” below).32,33
Hematopoietic cells are first seen in the marrow of the 10- to 11-week embryo,1 and they remain confined to the diaphyseal regions of long bones until 15 weeks’ gestation.34 Initially, there are approximately equal numbers of myeloid and erythroid cells in the fetal marrow. However, myeloid cells predominate by 12 weeks’ gestation, and the myeloid-to-erythroid ratio approaches the adult level of 3:1 by 21 weeks’ gestation.20 Macrophage cells in the fetal marrow, but not in the fetal liver, express the lipopolysaccharide receptor CD14.29 The marrow becomes the major site of hematopoiesis after the 24th week of gestation and remains so throughout the remainder of fetal life.
The reconstitution of the entire hematopoietic system by transplantation with cord blood indicates that hematopoietic stem cells are circulating in the blood at birth.35 The immunologic reconstitution of an immunodeficient human fetus with fetal-liver-derived cells also indicates that hematopoietic stem cells exist in the late gestation fetal liver.36 It was first postulated that hematopoietic stem cells originate independently in each hematopoietic site (yolk sac, liver, and marrow) of the embryo.37 However, experiments in the mammalian embryo indicate that the liver rudiment, like the marrow, is seeded by exogenous hematopoietic cells.38,39 It was initially thought that the liver, and eventually the marrow, were seeded by yolk sac–derived hematopoietic stem cells.40 However, experiments in avian and amphibian embryos indicate that the hematopoietic stem cells that ultimately provide for long-term adult hematopoiesis arise within the body of the embryo proper rather than from the yolk sac.41,42 Subsequent investigations in the mouse embryo indicate that stem cells capable of engrafting myeloablated adult recipients originate in the aorta-gonad-mesonephros (AGM) region of the embryo proper.43 Cells capable of long-term engraftment of immunodeficient mice also first originate at 35 days of gestation in the aorta region of human embryos.44 This correlates anatomically with the transient appearance of clusters of CD34+ blood cells closely associated with the ventral wall of the aorta in several mammalian species, including the 5 weeks’ gestation human embryo.45,46 These findings, as well as direct visualization of developing zebrafish embryos,47 support the concept that hematopoietic stem cells arise from “hemogenic” aortic endothelium through an endothelial-to-hematopoietic transition and then seed the liver, and eventually the marrow, to provide lifelong hematopoiesis (see Fig. 7–1). Studies in the murine embryo indicate that the placenta also serves as a site of hematopoietic stem cell origin and expansion.48 It is not known if the placenta serves a similar function during human development. The underlying relationship of the transient erythromyeloid hematopoiesis derived from the yolk sac to long-term hematopoietic stem cell–derived intraembryonic hematopoiesis remains unclear. However, studies in the mouse indicate that resident macrophage populations in multiple organs of the adult, particularly microglia in the brain, are ultimately derived from the yolk sac of the embryo and undergo limited maintenance after birth from hematopoietic stem cells.49,50
Human hemoglobin (Hgb) is a tetramer composed of two α-type and two β-type globin chains (Table 7–1). The α-globin gene cluster is located on chromosome 16 and contains the ζ gene 5′ to the pair of α-globin genes. The β-globin gene cluster is located on chromosome 11 and contains five globin genes oriented 5′ to 3′ as ε–γ A–γ G–δ–β.51 During embryogenesis the genes on both chromosomes are activated sequentially from the 5′ to the 3′ end. This globin “switching” is related not only to the relative positions of the globin genes within their respective chromosomal clusters, but also to interacting upstream “locus control regions.”52
Hgb Gower-1 (ζ2ε2) is the major hemoglobin in embryos younger than 5 weeks’ gestation (see Table 7–1).53 Hgb Gower-2 (α2ε2) has been found in embryos with a gestational age as young as 4 weeks and is absent in embryos older than 13 weeks.54 Hgb Portland (ζ2γ2) is found in young embryos, but persists in infants with homozygous α-thalassemia (Chap. 48). Synthesis of the ζ and ε chains decreases as those of the α and γ chains increase (Fig. 7–2). The ζ-to-α-globin switch precedes the ε-to-γ-globin switch as the liver replaces the yolk sac as the main site of erythropoiesis.9,55
Hgb F (α2γ2) is the major hemoglobin of fetal life (see Fig. 7–2).56 Synthesis of Hgb A can be demonstrated in fetuses as young as 9 weeks’ gestation.57 In fetuses of 9 to 21 weeks’ gestation, the amount of Hgb A (α2β2) rises from 4 to 13 percent of the total hemoglobin.57 These levels of Hgb A have enabled the antenatal diagnosis of β-thalassemia using globin-chain synthesis. After 34 to 36 weeks’ gestation the percentage of Hgb A rises, whereas that of Hgb F decreases (see Fig. 7–2). The mean synthesis of Hgb F in term infants was 59.0 ± 10 percent (1 SD) of total hemoglobin synthesis as assessed by 14C-leucine uptake.58 The amount of Hgb F in blood varies in term infants from 53 to 95 percent of total hemoglobin.59
The fetal hemoglobin concentration in blood decreases after birth by approximately 3 percent per week and is generally less than 2 to 3 percent of the total hemoglobin by 6 months of age. This rate of decrease in Hgb F production is closely related to the gestational age of the infant and is not affected by the changes in environment and oxygen tension that occur at the time of birth.60 Hgb A2 (α2δ2) has not been detected in fetuses. Normal adult levels of Hgb A2 are achieved by 4 months of age.61 Increased proportions of Hgb F at birth have been reported in infants who are small for gestational age, who have experienced chronic intrauterine hypoxia, who have trisomy 13, or who have died from sudden infant death syndrome (SIDS).62,63,64,65,66 Decreased levels of Hgb F at birth are found in trisomy 21.67
The cellular composition of fetal blood changes markedly during the second and third trimesters. The mean blood hemoglobin concentration in fetuses progressively increases from 9.0 ± 2.8 g/dL at age 10 weeks to 16.5 ± 4.0 g/dL at age 39 weeks.68 There is a concomitant decrease in the MCV of fetal red cells from a mean of 134 fL/cell at 18 weeks’ gestation to 118 fL/cell at 30 weeks’ gestation.69 The total white blood cell count averages 2 × 109/L between 10 and 17 weeks of gestation,31 and increases during the middle trimester to between 4.0 and 4.5 × 109/L, with an 80 to 85 percent preponderance of lymphocytes and 5 to 10 percent neutrophils.69 The percentage of circulating nucleated red cells decreases from a mean of 12 percent at 18 weeks to 4 percent at 30 weeks.69 The platelet count remains greater than 150,000/μL from 15 weeks’ gestation to term.69,70
Large numbers of committed hematopoietic progenitors circulate in the fetal blood. Blood samples obtained by fetoscopy at 12 to 19 weeks’ gestation reveal a mean of 20,450 BFU-E/mL and 12,490 CFU-GM/mL.71 This is in striking contrast to adult blood, which contains many fewer erythroid progenitors and 30 to 250 CFU-GM/mL.70,72 Most (70–80%) circulating hematopoietic progenitors at 26 to 28 weeks’ gestation are cycling.72 In contrast, adult-marrow-derived progenitors in the bloodstream are relatively quiescent with only 0 to 5 percent cycling.
NEONATAL HEMATOPOIESIS
The mean hemoglobin level in cord blood at term is 16.8 g/dL, with 95 percent of the values falling between 13.7 and 20.1 g/dL.73 This variation reflects perinatal events, particularly asphyxia,74 and also the amount of blood transferred from the placenta to the infant after delivery. Early cord clamping appears to heighten the occurrence of anemia at 2 months and to impair cardiopulmonary adaptation.75 Delay of cord clamping may increase the blood volume and red cell mass of the infant by as much as 55 percent.76,77 This results in fewer transfusions and fewer days requiring oxygen and ventilation in preterm infants.75 The mean total blood volume after birth is 86 mL/kg for the term infant and 89 mL/kg for the premature infant.78 The blood volume per kilogram decreases over the ensuing weeks, reaching a mean value of approximately 65 mL/kg by 3 to 4 months of age.
Normally the hemoglobin and hematocrit values rise in the first several hours after birth because of the movement of plasma from the intravascular to the extravascular space.79 A venous hemoglobin concentration of less than 14 g/dL in a term infant and/or a fall in hemoglobin or hematocrit level in the first postnatal day are abnormal. Table 7–2 shows the normal red cell values from capillary blood samples for term infants in the first 12 weeks after birth.80 Capillary hematocrit values in newborns are higher than those in simultaneous venous samples, particularly during the first postnatal days, and the capillary-to-venous ratio is approximately 1.1:1.81 This difference reflects circulatory factors and is greater in preterm and sick infants.
Age | Hbg, g/dL ± SD | RBC × 1012/L ± SD | Hematocrit, % ± SD | MCV, fL ± SD | MCHC, g/dL ± SD | Reticulocytes, % ± SD |
---|---|---|---|---|---|---|
DAYS | ||||||
1 | 19.3 ± 2.2 | 5.14 ± 0.7 | 61 ± 7.4 | 119 ± 9.4 | 31.6 ± 1.9 | 3.2 ± 1.4 |
2 | 19.0 ± 1.9 | 5.15 ± 0.8 | 60 ± 6.4 | 115 ± 7.0 | 31.6 ± 1.4 | 3.2 ± 1.3 |
3 | 18.8 ± 2.0 | 5.11 ± 0.7 | 62 ± 9.3 | 116 ± 5.3 | 31.1 ± 2.8 | 2.8 ± 1.7 |
4 | 18.6 ± 2.1 | 5.00 ± 0.6 | 57 ± 8.1 | 114 ± 7.5 | 32.6 ± 1.5 | 1.8 ± 1.1 |
5 | 17.6 ± 1.1 | 4.97 ± 0.4 | 57 ± 7.3 | 114 ± 8.9 | 30.9 ± 2.2 | 1.2 ± 0.2 |
6 | 17.4 ± 2.2 | 5.00 ± 0.7 | 54 ± 7.2 | 113 ± 10.0 | 32.2 ± 1.6 | 0.6 ± 0.2 |
7 | 17.9 ± 2.5 | 4.86 ± 0.6 | 56 ± 9.4 | 118 ± 11.2 | 32.0 ± 1.6 | 0.5 ± 0.4 |
WEEKS | ||||||
1–2 | 17.3 ± 2.3 | 4.80 ± 0.8 | 54 ± 8.3 | 112 ± 19.0 | 32.1 ± 2.9 | 0.5 ± 0.3 |
2–3 | 15.6 ± 2.6 | 4.20 ± 0.6 | 46 ± 7.3 | 111 ± 8.2 | 33.9 ± 1.9 | 0.8 ± 0.6 |
3–4 | 14.2 ± 2.1 | 4.00 ± 0.6 | 43 ± 5.7 | 105 ± 7.5 | 33.5 ± 1.6 | 0.6 ± 0.3 |
4–5 | 12.7 ± 1.6 | 3.60 ± 0.4 | 36 ± 4.8 | 101 ± 8.1 | 34.9 ± 1.6 | 0.9 ± 0.8 |
5–6 | 11.9 ± 1.5 | 3.55 ± 0.2 | 36 ± 6.2 | 102 ± 10.2 | 34.1 ± 2.9 | 1.0 ± 0.7 |
6–7 | 12.0 ± 1.5 | 3.40 ± 0.4 | 36 ± 4.8 | 105 ± 12.0 | 33.8 ± 2.3 | 1.2 ± 0.7 |
7–8 | 11.1 ± 1.1 | 3.40 ± 0.4 | 33 ± 3.7 | 100 ± 13.0 | 33.7 ± 2.6 | 1.5 ± 0.7 |
8–9 | 10.7 ± 0.9 | 3.40 ± 0.5 | 31 ± 2.5 | 93 ± 12.0 | 34.1 ± 2.2 | 1.8 ± 1.0 |
9–10 | 11.2 ± 0.9 | 3.60 ± 0.3 | 32 ± 2.7 | 91 ± 9.3 | 34.3 ± 2.9 | 1.2 ± 0.6 |
10–11 | 11.4 ± 0.9 | 3.70 ± 0.4 | 34 ± 2.1 | 91 ± 7.7 | 33.2 ± 2.4 | 1.2 ± 0.7 |
11–12 | 11.3 ± 0.9 | 3.70 ± 0.3 | 33 ± 3.3 | 88 ± 7.9 | 34.8 ± 2.2 | 0.7 ± 0.3 |
The red cells of the newborn are macrocytic, with an MCV in excess of 110 fL/cell. The MCV begins to fall after the first week, reaching adult values by the ninth week (see Table 7–2).80,82 The blood film from a newborn infant shows macrocytic normochromic cells, polychromasia, and a few nucleated red blood cells. Even in healthy infants there may be mild anisopoikilocytosis.83 Three to 5 percent of the red cells may be fragments, target cells, or otherwise distorted. By 3 to 5 days after birth, nucleated red blood cells are not found normally in the blood of term or premature infants, but they may be present in markedly elevated numbers in the presence of hemolysis or hypoxic stress. As expected from these findings, the red cell distribution width (RDW) is markedly elevated in the newborn period.84
There are significant numbers of circulating progenitor cells in cord blood.85,86,87 Cord blood BFU-E and colony-forming unit–erythroid (CFU-E) differentiate more rapidly than their adult counterparts.88 Furthermore, the proportion of cord blood hematopoietic progenitors in the mitotic cycle is approximately 50 percent, intermediate between the proportions found in fetal and adult progenitor cells.72,86
In several,89,90 but not all,91 studies, premature infants at birth had lower hemoglobin levels, higher reticulocyte counts, and higher nucleated red cell counts than did the term infants. The reticulocyte counts of premature infants are inversely proportional to their gestational age, with a mean of 8 percent reticulocytes evident at 32 weeks’ gestation and 4 to 5 percent at term.92 Infants who are small for their gestational ages have higher red cell counts, hematocrit levels, and hemoglobin concentrations as compared with infants whose size is appropriate for their gestational age.90,93
Erythropoietin is the primary regulator of erythropoiesis (Chaps. 32 and 33). Although erythropoietin is present in cord blood, it falls to undetectable levels after birth in healthy infants.94 Subsequently, the reticulocyte count falls to less than 1 percent by the sixth day after birth.80,95 The red cell, hemoglobin, and hematocrit values decrease only slightly during the first week, but decline more rapidly in the following 5 to 8 weeks (see Table 7–2),80 producing the physiologic anemia of the newborn. The lowest hemoglobin values in the term infant occur at approximately 2 months of age.82 When the hemoglobin concentration falls below 11 g/dL, erythropoietic activity begins to increase. Erythropoietin can be measured after the 60th postnatal day,96 corresponding to the recovery from physiologic anemia. If there is sufficient stimulus, such as hemolytic anemia or cyanotic heart disease, the newborn infant is able to produce erythropoietin prior to the 60th postnatal day.94
The fall in hemoglobin level is more pronounced in the premature infant. In one study of premature infants, the mean hemoglobin level at 2 months was 9.4 g/dL, with a 95 percent range of 7.2 to 11.7 g/dL.97 In healthy premature infants erythropoietin becomes detectable when the hemoglobin level falls to approximately 12 g/dL. In infants with a lower percentage of Hgb F (as from transfusion) and, consequently, better oxygen delivery, erythropoietin does not rise until the hemoglobin falls to approximately 9.5 g/dL.98 The mean values for iron-sufficient premature infants reached those of term infants by 4 months for red cell count, 5 months for hemoglobin level, and 6 months for mean corpuscular volume and mean corpuscular hemoglobin.97
The viscosity of blood increases logarithmically in relation to the hematocrit.99,100 Hyperviscosity was found in 5 percent of infants,101 and in 18 percent of infants who were small for gestational age.102 Newborn infants with hematocrit values of greater than 65 to 70 percent may become symptomatic because of increased viscosity.103 In one study of infants with documented hyperviscosity and a mean hematocrit greater than 65 percent, 38 percent displayed symptoms of irritability, hypotonia, tremors, or poor suck reflex.104 Partial plasma exchange transfusion reduced blood viscosity, improved cerebral blood flow, and relieved the symptoms. However, cerebral blood flow was normal in the asymptomatic infants with hyperviscosity, and, consequently, there was no benefit from exchange transfusion.104 Studies of neurodevelopmental status do not show any clear long-term benefits for the use of partial exchange transfusions in asymptomatic neonates.105
The blood group antigens on neonatal red cells differ from those of the older child and adult. The i antigen is expressed strongly, whereas the I antigen and the A and B antigens are expressed only weakly on neonatal red cells. The i antigen is a straight-chain carbohydrate that is replaced by the branched-chain derivative, I antigen, as a result of the developmental acquisition of a glycosyltransferase.106 By 1 year of age the i antigen is undetectable, and the ABH antigens increase to adult levels by age 3 years (Chap. 136). The ABH, Kell, Duffy, and Vel antigens can be detected on the cells of the fetus in the first trimester and are present at birth.107 The Lua and Lub antigens also are detectable on fetal red cells and are more weakly expressed at birth, increasing to adult levels by age 15 years.107 The Xg antigen is variably expressed in the fetus and is weaker on newborn than on adult red cells. Moreover, particularly poor expression of Xg has been noted in newborns with trisomy 13, 18, and 21.107 The Lewis group (Lea/Leb) antigens are adsorbed on the red cell membrane and become detectable within 1 to 2 weeks after birth as the receptor sites develop. Anti-A and anti-B isohemagglutinins develop during the first 6 postnatal months, reaching adult levels by 2 years of age.
The life span of the red cells in the newborn infant is shorter than that of red cells in the adult (Chap. 33). The average of several studies of mean half-life of newborn red cells is 60 to 80 days.108 The reasons for this shortened survival are unclear, but the known susceptibility to oxidant injury of newborn red cells may be a contributing factor.
The serum iron level in cord blood of the normal infant is elevated compared to maternal levels. The mean value is approximately 150 ± 40 mcg/dL (1 SD).109 Infants on an iron-supplemented diet have a median serum iron level of 125 mcg/dL at 1 month of age and of approximately 75 mcg/dL at 6 months of age. The total iron-binding capacity rises throughout the first year. The median transferrin saturation falls from almost 65 percent at 2 weeks to 25 percent at 1 year, and saturations as low as 10 percent may be observed in the absence of iron deficiency.110 The mean serum ferritin levels in iron-sufficient infants are high at birth, 160 mcg/L, rise further during the first month, and then fall to a mean of 30 mcg/L by 1 year of age.111 The amount of stainable iron in the marrow at birth is small but increases in both term and premature infants during the first weeks after birth. Stainable marrow iron begins to decrease after 2 months and is gone by 4 to 6 months in term infants and earlier in premature infants.112 Iron is preferentially allocated to erythropoiesis if the availability of iron is limited.113 This makes the availability of adequate iron particularly important to avoid iron lack in the brain, heart, and skeletal muscle.
The oxygen affinity of cord blood is greater than that of maternal blood, because the affinity of Hgb F for 2,3-bisphosphoglycerate (2,3-BPG) is less than that of Hgb A.114 Levels of 2,3-BPG are lower in newborn red cells than in adult cells and even more decreased in the red cells of premature infants,115 and this low 2,3-BPG level further heightens the oxygen affinity of newborn red cells. Consequently, the red cell oxygen equilibrium curve of the newborn is shifted to the left of that of the adult (Fig. 7–3). The mean partial pressure of oxygen (pO2) at which hemoglobin is 50 percent saturated with oxygen at 1 day of age in term infants is 19.4 ± 1.8 torr, as compared with the normal adult value of 27.0 ± 1.1 torr.116 This results in a decrease in the oxygen released at the tissue level, as shown in Fig. 7–3. As the partial pressure of oxygen (Po2) falls from 90 torr in arterial to 40 torr in the venous blood, 3.0 mL/dL of oxygen are released from newborn blood, whereas 4.5 mL/dL are released from adult Hgb A-containing blood. The shift to the left of the oxygen equilibrium curve is even more pronounced in the premature infant, requiring a larger fall in Po2 to release an equivalent amount of oxygen. After birth the oxygen equilibrium curve shifts gradually to the right, reaching the position of the adult curve by 6 months of age. The position of the curve in the premature infant correlates with gestational age rather than with postnatal age,116 and its shift to the adult position is more gradual.
Figure 7–3.
The oxygen equilibrium curves are based on the assumption that the Hgb concentration is 15 g/dL and that there is full O2 saturation of Hgb at a partial pressure of arterial oxygen (Pao2) of 100 torr. The O2 released is the difference in O2 content between a Pao2 of 90 torr and the mixed venous Pao2 of 40 torr. The O2 available is the difference in O2 content between a Pao2 of 90 torr and a mixed venous Pao2 of 20 torr. This is the maximum O2 available without evoking compensatory mechanisms such as increased cardiac output.
Many differences have been found between the metabolism of the red cells of newborn infants and that of adults.117,118 Some of the differences may be explained by the younger mean cell age in the newborn, but others seem to be properties of the fetal cell. The glucose consumption in newborn red cells is lower than that in adult red cells.119 Elevated levels of glucose phosphate isomerase, glyceraldehyde-3-phosphate dehydrogenase, phosphoglycerate kinase, and enolase beyond those explainable by the young cell age have been found in neonatal cells.115,120 The level of phosphofructokinase is low in red cells of term and premature infants.115,120,121 The pentose phosphate shunt is active in red cells of term and premature infants,122 but glutathione instability leads to a heightened susceptibility to oxidant injury. The result of oxidant stress is depletion of adenosine triphosphate (ATP) and adenine nucleotides leading to iron release, denaturing of membrane proteins, and hemoglobin and membrane peroxidation.123 The levels of ATP and adenosine diphosphate (ADP) are higher in the red cells of term and preterm infants,121 but may merely reflect the younger age of the erythrocyte population. Finally, lower-than-adult activities have been found for several other red cell enzymes, including cytochrome B5 reductase124 and glutathione peroxidase.125
The membrane of the newborn red cell also is different from that of the adult red cell. Ouabain-sensitive adenosine triphosphatase (ATPase) is decreased,126 and active potassium influx is significantly less in neonatal red cells.127 Newborn cells are more sensitive to osmotic hemolysis and to oxidant injury than are adult cells. Newborn red cell membranes have higher total lipid, phospholipid, and cholesterol per cell than adult red cells.128,129 The patterns of phospholipid and phospholipid fatty acid composition also differ from those in adult red cells. Red cells of newborns have the same pattern of membrane proteins on polyacrylamide gel electrophoresis130 and the same rate of mobility in an electric field131 as do red cells from adults. After trypsin treatment of newborn and adult cells, however, there is a difference in electrophoretic mobility, indicating that the surface trypsin-resistant proteins are different.131 The relationship of the metabolic and membrane alterations in neonatal red cells to their shorter life span is not clear.
The absolute number of neutrophils in the blood of term and premature infants usually is greater than that found in older children (Table 7–3).132 The neutrophil count tends to be lower in the premature than in the term infant, and the proportion of myelocytes and band neutrophils is higher.133 Serum and urinary colony-stimulating activity are elevated during the period of neutrophilia.134 When granulopoiesis was studied in cord blood, blood, and marrow of infants, the macrophage colony-forming unit was predominant despite the clinical neutrophilia, and this pattern was not altered by different sources of colony-stimulating factors.135,136 The endogenous cytokines produced by mononuclear cells from cord or systemic venous blood support the growth of neutrophil colonies in assays using marrow from adults.135 However, there is diminished GM-CSF, G-CSF, and IL-3 production in stimulated newborn compared to adult mononuclear cells,137,138,139 which may limit the response to bacterial infection in the newborn. Furthermore, preterm infants have a reduced neutrophil storage pool and a restricted capacity to increase their progenitor proliferation, and their neutrophil count may fall precipitously with neonatal bacterial infection.140 Dysregulation, as well as diminished capacity of neonatal granulopoiesis, may impair the neonatal response to infection.141 Smaller numbers of CFU-GM colonies were observed in the blood of sick infants, who also have diminished endogenous production of colony-stimulating factors in culture.136 The clinical use of cytokines to treat neonatal sepsis remains controversial,142 but circulating neutrophils are increased in preterm infants treated with recombinant G-CSF, and the infants’ length of stay in the neonatal intensive care unit is shortened.143
Neutrophils | ||||||||
---|---|---|---|---|---|---|---|---|
Age | Leukocytes | Total | Segmented | Bands | Eosinophils | Basophils | Lymphocytes | Monocytes |
BIRTH | ||||||||
Mean | 18.0 | 11.0 | 9.4 | 1.6 | 0.40 | 0.10 | 5.5 | 1.05 |
Range | 9.0–30.0 | 6.0–26.0 | — | — | 0.02–0.85 | 0–0.64 | 2.0–11.0 | 0.4–3.1 |
Mean % | — | 61 | 52 | 9 | 2.2 | 0.6 | 31 | 5.8 |
7 DAYS | ||||||||
Mean | 12.2 | 5.5 | 4.7 | 0.83 | 0.50 | 0.05 | 5.0 | 1.1 |
Range | 5.0–21.0 | 1.5–10.0 | — | — | 0.07–1.1 | 0–0.25 | 2.0–17.0 | 0.3–2.7 |
Mean % | — | 45 | 39 | 6 | 4.1 | 0.4 | 41 | 9.1 |
14 DAYS | ||||||||
Mean | 11.4 | 4.5 | 3.9 | 0.63 | 0.35 | 0.05 | 5.5 | 1.0 |
Range | 5.0–20.0 | 1.0–9.5 | — | — | 0.07–1.0 | 0–0.23 | 2.0–17.0 | 0.2–2.4 |
Mean % | — | 40 | 34 | 5.5 | 3.1 | 0.4 | 48 | 8.8 |
Table 7–3 gives the values for the white cell and differential counts during the first 2 weeks after birth. The absolute number of segmented neutrophils rises in both term and premature infants in the first 24 hours.144 In term infants, the mean value increases from 8 × 109/L to a peak of 13 × 109/L and then falls to 4 × 109/L by 72 hours of age, remaining at this level through the following 7 days. In the premature infant, the mean values for neutrophils are 5 × 109/L at birth, 8 × 109/L at 12 hours, and 4 × 109/L at 72 hours. The mean count then falls gradually to 2.5 × 109/L by the 28th postnatal day. The level after the first 72 hours is very stable for an individual infant, whether term or premature. Immature forms, including an occasional promyelocyte and blast cell, may be seen in the blood of healthy infants in the first few days after birth and are more frequent in premature infants than in term infants.144 Segmented granulocytes are the predominant cells in the first few days after birth. As their number decreases, the lymphocyte becomes the most numerous cell and remains so during the first 4 years of life. An absolute eosinophil count of greater than 0.7 × 109/L was found in 76 percent of premature infants at 2 to 3 weeks of age. The onset of the eosinophilia coincided with the establishment of steady weight gain in the infants.145 It is increased by the use of total parenteral nutrition, endotracheal intubation, and blood transfusions.
Bacterial infections are a major cause of morbidity and mortality in the newborn period.146 The infections frequently are caused by organisms of low virulence in normal children and adults, including Staphylococcus and Lancefield group B β-hemolytic streptococci, but, also, by Pseudomonas, and other Gram-negative bacilli. Cellular defense mechanisms and humoral immunity of the newborn differ from those found later in life, and these undoubtedly contribute to the unusual susceptibility to infection noted in the neonatal period.146
Engulfment and destruction of bacteria by neutrophils depend on opsonic activity of the plasma and on chemotaxis, phagocytosis, and the bacteriocidal capacity of the leukocyte. The serum factors necessary for optimal phagocytosis (opsonins) include the immunoglobulins and complement components. In term infants, opsonic activity is normal for Staphylococcus aureus,147,148 but it is low for yeast149 and Escherichia coli.147,148 Diminished opsonic antibody is associated with group B streptococcal infection and represents one risk factor for neonatal infection.150
In premature infants, opsonic activity is low for S. aureus and Serratia marcescens,147 but is normal for Pseudomonas aeruginosa.151 When serum concentrations of fibronectin and IgG subclasses C3 and C4 were measured at birth, 1 month, 3 months, and 6 months, early gestational age was correlated with lower initial levels.152 The decreased opsonic activity for some organisms in premature infants is attributed to diminished IgG levels, because additional IgG will correct the opsonic defect both in vivo and in vitro.147
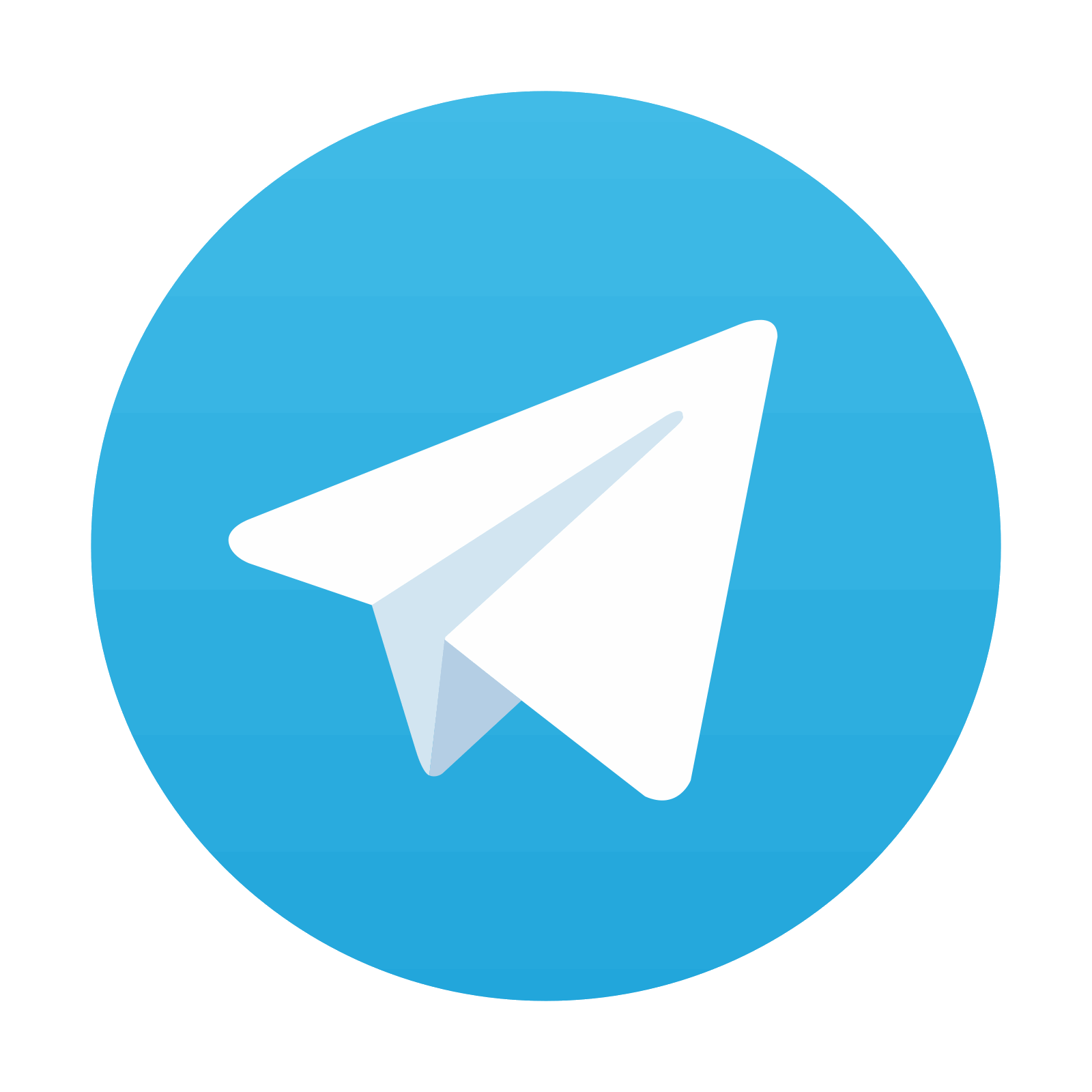
Stay updated, free articles. Join our Telegram channel
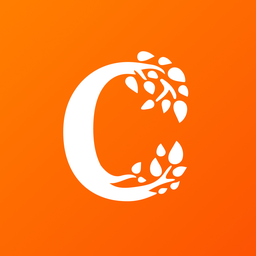
Full access? Get Clinical Tree
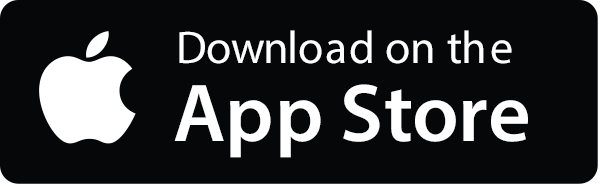
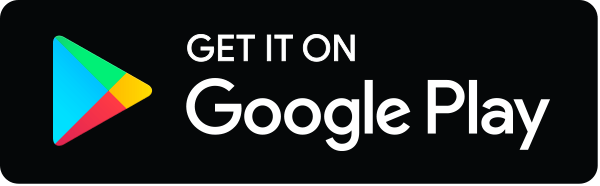
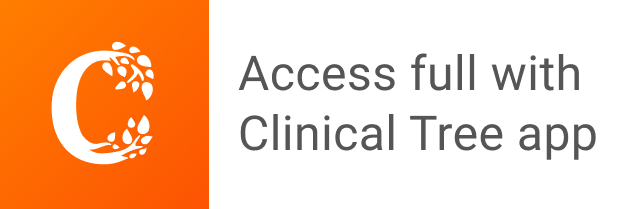