INTRODUCTION
SUMMARY
Production of red cells or erythropoiesis, is a tightly regulated process by which hematopoietic stem cells differentiate into erythroid progenitors and then mature into red cells. Erythropoiesis generates approximately 2 × 1011 new erythrocytes to replace the 2 × 1011 red cells (approximately 1 percent of the total red cell mass) removed from the circulation each day. Red cell production increases several fold after blood loss or hemolysis. When one of the progeny of the multipotential hematopoietic stem becomes committed to the erythroid lineage, this early erythroid progenitor undergoes a series of divisions and concurrent maturation that eventually result in morphologically recognizable erythroblasts. After expulsion of the nucleus, a macrocyte (polychromatophilic when stained by Wright stain, or a reticulocyte if stained with new methylene blue) leaves the marrow. During the first 24 hours in the circulation, reticulocytes lose their residual organelles (mitochondria and ribosomes) through an autophagic process and undergoes reconditioning of the membrane to become mature red blood cells with a morphology of a biconcave disc. Erythropoiesis is controlled by transcription factors and cytokines, the principal ones being GATA 1 and erythropoietin (EPO), which influence the rate of lineage commitment, proliferation, apoptosis, differentiation, and number of divisions from the earliest progenitor to late erythroblasts. The number of red cells produced varies in response to tissue oxygenation that determines the level of the transcription factors, hypoxia-inducible factors (HIF), HIF-1 and HIF-2, the principal regulators of the response to hypoxia. HIFs modulate erythropoiesis by regulation of EPO production, by direct EPO-independent mechanism(s) and facilitating iron availability.
Acronyms and Abbreviations:
BCL11A, a critical switching factor for silencing γ-globin; Bcl-xL, an antiapoptotic factor; BFU-E, burst-forming units–erythroid; CBP, a coactivator of a transcription factor; CFU-E, colony-forming units-erythroid; CFU-Ec-Kit, growth factor receptor also a protooncogene; CIS, a signal transduction protein that downregulates activity of erythropoietin receptor; CPM, counts per minute; EKLF, erythroid Kruppel-like factor; Emp, erythroblast-macrophage protein; EPO, erythropoietin; EPOR, EPO receptor; FOG, “friend of GATA,” a GATA-1 interacting protein; Gas6, growth arrest-specific 6; GATA-1, transcription factor; HCP, hematopoietic cell phosphatase; Hct, hematocrit; HIF, hypoxia-inducible transcription factor; ICSH, International Committee on Standardization in Hematology; JAK2, a tyrosine kinase that interacts with erythropoietin receptor; KAP1, KRAB-associated protein-1 is a transcriptional cofactor; KRAB-ZFP, one of the 400 human zinc finger protein-based transcription factors; mDia2, a protein that regulates actin and focal adhesion dynamics; miRNAs, microRNAs are small noncoding RNA molecules; NFE-2, a transcription factor, one of the principal regulator of hematopoiesis; Nix, a protein that is expressed during erythropoiesis and regulates mitochondrial apoptosis (autophagy); OS-9, osteosarcoma protein 9; PU.1, a transcription factor; RACK1, receptor of activated protein kinase C; RCM, red cell mass; SCL/TAL1, stem cell leukemia/T-cell acute lymphoblastic leukemia 1 factor; SOCS3, a signal transduction protein (also known as CIS3) that downregulates activity of erythropoietin receptor; VHL, von Hippel-Lindau protein.
HISTORY
Erythrocytes evolved largely for the purpose of transporting oxygen to tissues. Thus, the size of the red cell mass and the rate of red cell production must be closely related to supply and demand for oxygen in the tissues. Toward the end of the 19th century, French mountaineers and physiologists established that a low tissue tension of oxygen stimulates red cell production.1 In 1906, Paul Carnot, a professor at the Sorbonne, and Mademoiselle DeFlandre, his associate, suggested that hypoxia generates a humoral factor capable of stimulating red cell production.2 Based on questionable experimental data, an influential biochemist Friederich Miescher3 erroneously proposed that marrow hypoxia directly stimulates red cell production. Finally, in 1950, in an ingenious study on parabiotic rats, Kurt Reissmann4 provided evidence for the existence of an indirect humoral mechanism. This work, and work of Erslev and colleagues5,6 who demonstrated that the plasma from anemic rabbits and primates contains an erythrocyte-stimulating factor, provided a strong basis for an existence of the factor appropriately named erythropoietin (EPO). In 1957, Jacobson and coworkers7 reported that EPO was produced by the kidney, a finding that raised the possibility that EPO isolated in adequate amounts might be of therapeutic benefit to uremic patients. After EPO cloning and production of recombinant EPO in therapeutic quantities, EPO has proved to have not only indications for therapy of anemia, but also has extraerythroid effects that are yet to be fully elucidated, such as its effect on cell growth. Widespread use of EPO for the treatment of anemia has surpassed original expectations.
PHYLOGENY OF RED CELL PRODUCTION
Hemoglobin is present in the most primitive animal forms, such as Paramecium and Tetrahymena. Some crustaceans, such as Daphnia, are capable of developing an oxygen transport system without circulating red cells.8 One interesting exception is an Antarctic ice fish (Chaenocephalus aceratus) lacking hemoglobin.9 These ice fish compensate for the absence of hemoglobin by their unusual nitric oxide metabolism.10,11,12 They have very large hearts and unusually large diameter capillaries. This permits a large volume of blood to circulate at high flow rate and at low vascular pressure because of decreased peripheral resistance. This permits their survival in the very high oxygen content of Antarctic waters.12
An erythroid cell that can synthesize, carry, and protect hemoglobin from oxidation was found only with the development of a circulatory system. Circulating nucleated erythrocytes first appear in the worms of the phylum Nemertina and in the sessile marine creatures of the phylum Phoronida. Erythropoiesis in these primitive invertebrates takes place near or on the peritoneal surface, derived from endothelial cells.13 Nonnucleated red cells are observed for the first time in the more advanced phylum Annelida. However, the evolutionary advantage derived from enucleation appears to be slight. Nucleated red cells are observed in more advanced animals, such as reptiles and birds.14 All mammalian erythrocytes are nonnucleated and in most species are disc shaped, but are oval in some species.15 Enucleation decreases the workload of heart as it reduces one third of the cell weight.
In nonmammalian species, the spleen is the fundamental erythropoietic organ. However, in some fish, the kidneys also are involved in red cell production.16,17 In vertebrates, an evolutionary shift occurred from the spleen to the liver and from the liver to the bones cavities.18 The homeostatic regulation of blood or hemoglobin production has been studied in Daphnia,8 where a balance exists between oxygen need and hemoglobin production. In higher animals, this relationship is maintained by adjusting red cell production. Studies of birds,19 fish,20 and mammals21 indicate that red cell production is controlled by EPO, which is capable of adjusting red cell production to the demands for oxygen in the tissues. EPO of mammals has considerable biologic similarity and genetic homology.22
ONTOGENY OF RED CELL PRODUCTION
The environment within the bone apparently is optimal for cellular proliferation and maturation. However, bone cavities do not develop until the fifth fetal month. Other, presumably less favorable, sites are responsible for red cell production during early embryonic life (Chap. 7). In the human, large nucleated blood cells are first formed in the yolk sac,23 and some enucleate.24 They cluster in blood islands that become enveloped by endothelial cells forming the vascular plexus of the yolk sac.25 This is referred to as primitive erythropoiesis, and is contrasted with definitive erythropoiesis, which occurs in the fetal liver and in the marrow. During the second gestational month, erythropoiesis moves to fetal liver, wherein smaller, but still macrocytic, nonnucleated cells are produced.26,27 At birth, the hepatic phase of blood cell production ceases, and erythropoiesis moves to the marrow (Chaps. 7 and 48 provide details of developmental switching of embryonic, fetal, and adult globin expression).
During the neonatal period, the volume of available marrow space is almost the same as the total volume of hematopoietic cells and marrow vasculature.28 This process continues for a few years until the growth of bones and bone cavities exceeds the growth of hematopoietic mass. However, whenever the demand on erythropoiesis increases (blood loss, hypoxia, ineffective erythropoiesis, or hemolysis), the lack of reserve space in neonates and small children reactivates extramedullary erythropoiesis in the liver and spleen.29 In adults, expansion of marrow space continues, and the amount of fatty tissue gradually increases in all bone cavities. Because of the abundant marrow space, compensatory reactivation of extramedullary sites rarely occurs in later life. Extramedullary hematopoiesis during adult years indicates pathologic rather than compensatory blood formation, such as seen in primary myelofibrosis (Chap. 86) wherein the stem cells have abnormal interaction with the extracellular matrix.30 During fetal life, EPO production is primarily hepatic.31 At birth, a gradual switch to renal production of EPO occurs. In the adult, the kidney is responsible for approximately 85 percent of total production.32,33
CELLULAR COMPONENTS OF ERYTHROPOIESIS
Our ability to evaluate early erythropoiesis rests on functional assays of hematopoietic progenitors. The developmentally earliest progenitor committed to the erythroid lineage is the burst-forming unit–erythroid (BFU-E). It was initially termed a burst because it contains cells still capable of migration. These cells form smaller clusters around a larger central colony, giving the appearance of a sunburst with satellite colonies (Fig. 32–1). However, all the cells in the colony and its satellites are derived from a single BFU-E and, thus, are clonal. BFU-E takes longer than more mature erythroid progenitors to form a colony of erythroblasts (10 to 14 days) and form a large colony approximately 2000 to 3000 cells. BFU-E express low levels of EPO receptors (EPORs). BFU-E mature into colony-forming unit–erythroid (CFU-E), the more mature erythroid progenitor. A CFU-E is identified through more differentiated erythroid progenitor that in vitro form smaller colonies (50 to 200 cells) that mature in 3 to 5 days with EPOR density and EPO dependency increase gradually as progenitor cells mature, culminating at the level of the CFU-E.34,35 BFU-E and CFU-E cannot be identified by microscopy (Chap. 31), but they can be studied in vitro by their ability to generate microscopically recognizable hemoglobinized precursors (i.e., erythroblasts) by so-called clonogenic assays on semisolid media.
Figure 32–1.
Burst-forming unit–erythroid (BFU-E) and colony-forming unit–erythroid (CFU-E). Erythroid colony growth in methylcellulose medium in presence of erythropoietin. Normal human marrow. The colonies are stained for hemoglobin. A. BFU-E. This colony grows from a single marrow erythroid progenitor cell (BFU-E). It was photographed at 14 days in culture. The BFU-E is a differentiated cell, committed to the erythroid lineage. The BFU-E is a more primitive progenitor in the erythroid maturation pathway than the CFU-E. The colony it forms is large, compared to the CFU-E, has spreading margins, and often satellite colonies. B. CFU-E. This colony was photographed at day 7 in culture. The CFU-E originates from a more mature single progenitor cell than the BFU-E. The CFU-E is smaller and grows typically in a tight, dense colony, compared to the BFU-E. The sequence established in the erythroid lineage is BFU-E, CFU-E, erythrocyte precursors (proerythroblast, etc). (Reproduced with permission from Lichtman’s Atlas of Hematology, www.accessmedicine.com.)
In contrast, cells that constitute the latter stages of erythropoiesis can be identified by light microscopy (Chap. 31). The earliest morphologically recognizable erythroid precursor in the adult marrow is the pronormoblast. Pronormoblasts are conspicuously large and they have large uncondensed nuclei and deep basophilic cytoplasm as a result of the presence of numerous RNA-containing polyribosomes. Pronormoblast has a volume of 900 fL, 10 times the volume of the mature red blood cell. With each successive division, the precursor cells give rise to daughter cells of half their volume. Furthermore, with each division there is an increase in hemoglobin synthesis and condensation of nucleus. Thus, when the pronormoblasts divide to become basophilic normoblasts, the daughter cells have less blue cytoplasm because of hemoglobin synthesis and also a greater condensation of the nucleus. When the basophilic normoblasts divide further, they give rise to mature cells with more cytoplasmic hemoglobin that is stainable with both acid and basic dyes resulting in muddy-colored cytoplasm. These cells are called polychromatophilic normoblasts. The offspring of polychromatophilic normoblasts are called orthochromic normoblasts. Their nuclear chromatin is completely condensed and cytoplasm is pink from complete hemoglobinization. These cells do not divide further. Following extrusion of the nucleus, the enucleated cells derived from orthochromic orthochromatic erythroblasts are termed reticulocytes, named after the cytoplasmic remnants of the endoplasmic reticulum and the persistence of a few mitochondria and strings of ribosomes seen when stained with supravital dyes. Reticulocytes remain in the marrow for 48 to 72 hours before being released to the blood. The reticulocytes have an irregular polylobated shape and various membrane-bound organelles.36 In the blood, immature erythrocytes (reticulocytes) undergo further maturation with the removal of vestiges of organelles and reconditioning of the membrane to become mature red blood cells with the morphology of a biconcave disk.37
The number of erythroid precursor cells determines to a great extent the number of red cells produced. The proerythroblasts also contain EPORs that, in the presence of higher than normal levels of EPO, may accelerate their entry into their first mitotic division. This process may lead to a shortened marrow transit time of erythroblasts38 and result in release of still immature erythrocytes (polychromatophilic macrocytes), so-called stress reticulocytes (Fig. 32–2).39 Creation of a normal sized and shaped red cells, devoid of organelles, is the end result of an orderly transformation of a proerythroblast with a large nucleus and a volume of approximately 900 fL to a hemoglobinized anucleate disc-shaped cell with a volume of approximately 90 fL. Although cytoplasmic maturation is continuous, the interposed mitotic divisions cause a stepwise reduction in cytoplasmic and nuclear volumes, enabling recognition of proerythroblasts, erythroblasts, and polychromatophilic macrocytes (reticulocytes) with light microscopy (Chap. 31). Direct measurements of the number of marrow erythroblasts and reticulocytes have shown approximately 50 erythroblasts and approximately 124 reticulocytes for each proerythroblast (Table 32–1).40,41 This distribution conforms to the number of cells in a theoretic erythroid pyramid (Table 32–1, Fig. 32–3). In the pyramid, each erythroblast undergoes five mitotic divisions over 5 days before the orthochromatic erythroblast loses its nucleus and as an immature erythrocyte enters a 2- to 3-day period of maturation before its release from the marrow. The size and shape of these erythroid pyramids undoubtedly vary, but such variations play a role in the physiologic control of red cell production. When production is suppressed, as in low EPO as seen in anemia of chronic renal disease, the distribution of erythroblasts appears normal, with no morphologic or ferrokinetic evidence of ineffective erythropoiesis but the number of erythroid progenitors is decreased.38 When production is increased, as in severe hemolytic anemia, the pyramid of erythroid precursors also appear normal, with no evidence of additional mitotic divisions but the number of erythroid progenitors is increased. Consequently, the rate of red cell production largely depends on the number of erythroid progenitors formed.
Figure 32–2.
Stress reticulocytes. A. Blood film. Hemolytic anemia. The polychromatophilic macrocyte with puckering evident by the cloverleaf-shaped clear areas (folds) is a characteristic stress erythrocyte, so named because they are prematurely released from the marrow by high levels of erythropoietin, usually as a result of a hemolytic anemia. They are large, intensely polychromatophilic, and often have evidence of excess surface area as evident by folds. B. Phase-contrast microscopy of the blood cells in suspension from a case of hemolytic anemia. The arrows point to two macrocytes with puckered (folded) surfaces, characteristic of stress reticulocytes. C. A scanning electron micrograph of a stress reticulocyte. Note the markedly increased surface area to volume relationship for a red cell. D. Scanning electron micrograph of a marrow sinus of a mouse. L denotes the sinus lumen. The asterisk is the edge of the endothelial lining of the sinus, torn in preparation for microscopy. The arrow points to two anucleate red cells folded amidst the reticular cell extensions that make up the stroma of marrow. Note the severe folding of reticulocytes in situ. Note similarity between folds of the cell to the scanning image in (C). Just below the asterisk is an enucleated red cell (reticulocyte) half in the hematopoietic space and half in the lumen, presumptively in egress. Note the surface folding required when traversing the narrow pore in endothelium. E. A marrow sinus with an anucleate red cell emerging into the lumen. Note the folding required to negotiate the narrow pore through which the cell is exiting. (Chapter 3 provides details of erythrocyte egress.) (Reproduced with permission from Lichtman’s Atlas of Hematology, www.accessmedicine.com.)
As the erythroblast matures, its synthetic activities increase rapidly, producing all proteins characteristic of mature red blood cells, particularly globin. Eventually 95 percent of all protein in the red cell is hemoglobin, almost all hemoglobin A (α2β2) in adults, with only small amounts of hemoglobin F (α2γ2) and hemoglobin A2 (α2δ2). Hemoglobin F is unequally distributed and is present only in some erythrocytes, designated as F cells (Chaps. 48 and 49).
EPOR density declines sharply on early erythroblasts, and EPORs are absent from the more mature erythroblast forms while the number of receptors for transferrin increases, reflecting the increased demands for iron for heme synthesis.
The microenvironment may be important for proliferation and maturation of erythroblasts. However, in situ secreted or circulating growth factors and cytokines appear to be less important for precursor cells than for progenitor cells. Intercellular adhesion molecules secure the structural integrity of the marrow, and fibronectin is of special importance for erythroblasts.42 Loss of fibronectin receptors heralds the translocation of polychromatophilic macrocytes (reticulocytes) into blood, but some newly emerging erythrocytes remain sticky even after release and are temporarily sequestered by the spleen (Chap. 6). Because erythroid colonies developed in vitro consist principally of nucleated red cells, enucleation may primarily be induced by marrow stromal cells (Chaps. 5 and 31).
The extrusion of the spent pyknotic nuclei at terminal erythroid maturation is unique to mammals. This process results in the formation of pliable biconcave disc from rigid spheroidal cells. Enucleation decreases workload of the heart as it reduces one-third of the erythrocyte weight. The retinoblastoma protein and its effector, E2f-2, are critical for erythroid cells to exit the cell cycle for enucleation to take place.43 During terminal differentiation, the plasma membrane forms an envelope around the nucleus, followed by the formation of the contractile actin ring on the plasma membrane to move the nucleus for disposal.44 Rac guanosine triphosphatases (GTPases) and their effector mDia2 are required for the contractile actin ring movement in the release of the nucleus.45 After separation from the cell, the expelled nucleus displays phosphatidylserine and is recognized and engulfed by macrophages.46 Emp, an erythroblast–macrophage protein initially identified as a mediator of erythroblast–macrophage interactions, also plays a role in the enucleation.47 Emp associates with F-actin, an interaction important for the normal distribution of F-actin in both erythroblasts and macrophages. Emp null mice do not extrude their nuclei from erythroid cells. Thus, Emp appears to be required for erythroblast enucleation.48
Microscopic determination of marrow cellularity and proportion of erythroblasts permits semiquantitative evaluation of erythropoiesis. However, the presence of ineffective erythropoiesis in disease states, such as iron deficiency, anemia of chronic disease, megaloblastic anemias, and thalassemias, makes the morphologic approach misleading (Chaps. 37, 41, 42, and 48). Red cell production can be accurately estimated by ferrokinetic studies using 59Fe. Similarly, the amount of the final product of erythropoiesis, the red cell mass, can also be accurately measured. Unfortunately, the ever-increasing regulation of even minute amounts of radioisotopes used in vivo makes these methods available in only a few specialized centers.
Chapters 7, 30, 47, and 48 discuss developmental control of erythropoiesis, differential use of enzyme and globin genes, and the crucial differences between embryonic yolk sac and fetal/adult definite erythropoiesis. This chapter focuses mainly on adult erythropoiesis.
REGULATION OF ERYTHROPOIESIS
Erythropoiesis is a tightly regulated system, but the details are still not fully elucidated. Much remains to be learned from uncovering the molecular basis of many congenital and acquired mutations that disrupt the control of erythropoiesis. Two competing hypothesis have been proposed to explain the differentiation of the hematopoietic progenitors cells toward erythroid lineage.
According to the deterministic model of lineage commitment, specific extracellular signals, such as cytokines, play an instructive role in lineage specification.
Multipotential progenitors (Chap. 18) and erythroid unipotential progenitors, the BFU-E, require stem cell factor, interleukin-3, granulocyte-macrophage colony-stimulating factor, and/or thrombopoietin for growth and survival (Fig. 32–4).
Figure 32–4.
A. Cytokine influence on hematopoiesis. CFU-GEMM, colony-forming unit–growing granulocyte, erythrocyte, megakaryocyte, and macrophage precursors; G-CSF, granulocyte colony-stimulating factor; IL3, interleukin-3; SCF, stem cell factor; TPO, thrombopoietin. B. Regulation of erythropoiesis by hypoxia. HIF-1 hypoxia inducible factor-1; VEGF, vascular endothelial growth factor 1.
In contrast, the stochastic model proposes that spontaneous formation of a set of transcription factors, independently of extrinsic signals, mediates lineage commitment. These transcription factors activate a unique set of genes for a particular lineage and repress the action of alternative transcription factors and cytokines play only a permissive role. Most of evidence based on gene targeting studies and in vitro culture studies support the stochastic model of differentiation. Several transcription factors, such as GATA-1, FOG1, erythroid Kruppel-like factor (EKLF), PU.1, and SCL/TAL1 (stem cell leukemia/T-cell acute lymphoblastic leukemia 1 factor) have been characterized that are involved in erythroid differentiation.
The GATA family of zinc-finger transcription factors was first identified as the nuclear factors that bind to the GATA sequence in the enhancer region of the globin genes.49,50 GATA-1 protein is expressed during erythroid differentiation, with highest expression in CFU-Es and pronormoblasts. GATA-1 promotes erythroid differentiation by activating several erythroid-specific genes and represses transcription of Kit receptor and GATA-2. GATA-1 deficient mice die at embryonic day 10.5 with severe anemia from maturation arrest at the stage of pronormoblasts.51 In vitro, GATA-1 null the embryonic stem cells fail to mature beyond pronormoblast and undergo apoptosis. GATA-1 and its cofactor CBP are essential for the formation of an erythroid-specific histone acetylation pattern of histones at the active globin genes and the β-globin locus control region.52 GATA-1, along with EPO, induces expression of the antiapoptotic protein Bcl-xL53 and interacts with multiple proteins, including FOG-1 and PU.1,54 and FOG-1 acts as a cofactor for GATA-1.55 GATA-1 interaction with PU.1 appears to counteract erythropoiesis by inducing differentiation of pluripotent stem cell to myeloid and B lymphopoiesis and inhibition of erythropoiesis.54,56,57 Whereas PU.1 absence appears to be required for completion of terminal erythroid differentiation, low levels of PU.1 expression are essential for fetal erythropoiesis and for proper augmentation of adult erythropoiesis at times of stress.58
FOG-1, a member of friend of GATA family of zinc finger proteins, act as cofactors for GATA-1. It was first identified in a yeast two-hybrid screen for GATA-1 interacting proteins.55 It binds to the amino zinc finger of GATA-1. FOG-1−/− mice die during embryonic days 10.5 to 11.5 from severe anemia with arrest in erythroid maturation at a stage similar to that observed in the GATA-1− mice.59 FOG-1 physically interacts with GATA-1 to augment or inhibit its transcriptional activity depending on the promoter context.
GATA-2 was initially cloned as a GATA motif-binding factor is present in all erythroid cells; and, targeted deletion of GATA-2 resulted in embryonic lethality at day 10.5 from ablation of blood cell development.60 GATA-1 and GATA-2 directly regulate GATA-2 transcription in a reciprocal fashion during erythroid differentiation.61,62 GATA-2 autoregulates its transcription by binding to its own regulatory elements in the promoter region. This autoregulation is abolished by the displacement of GATA-2 by GATA-1 (GATA-2/GATA-1 switch), an interaction facilitated by FOG-1.63 Chromatin immunoprecipitation studies indicate that FOG-1 facilitates occupancy by GATA-1 at selected cis-regulatory chromatin elements. Double knockout of GATA-1 and GATA-2 results in embryonic lethality with complete absence of primitive erythropoiesis.64 The severity of this phenotype compared to either single GATA-1 or GATA-2 knockout suggests overlapping functions of these two transcription factors in primitive erythropoiesis.
EKLF is a zinc finger protein identified by subtractive hybridization of the mRNA of erythroid cells with common messages in a myeloid cell line.65 It interacts with CACCC sequence in the β-globin promoter, where it modifies chromatin structure permitting β-globin gene transcription. EKLF-deficient mice die at embryonic day 14.5 to 15 from severe anemia from defective definitive erythropoiesis.66 There is a marked decrease in β-globin mRNA and protein levels in EKLF-deficient erythroid cells. Large amounts of iron accumulate in the reticuloendothelial system of EKLF-deficient mice, consistent with an ineffective erythropoiesis.
SCL/TAL1 is a member of basic helix-loop-helix transcription factors essential for maturation of the erythroid and megakaryocytic lineages.67 Knockout of SCL/TAL1 leads to failure of hematopoiesis.68 Selective rescue of SCL/TAL1 null embryonic stem cells under the control of stem cell enhancer revealed differentiation blocks in erythroid and megakaryocytic maturation.69 Conditional knockout studies have revealed that erythroid and megakaryocytic precursors do not develop in the marrow of mice upon deletion of SCL/TAL1.70 Heterodimerization of SCL with other transcription factors, such as E2A, is a prerequisite for its functions.71
BCL11A, a transcription factor initially identified in lymphoid cells, regulates erythroid differentiation, especially in switching from fetal to adult hemoglobin.71 Fetal hemoglobin (HbF) levels decline after birth and are then replaced by adult hemoglobin A. The molecular mechanisms responsible for this switch are not completely known. Genome-wide association findings have provided a major breakthrough in understanding this phenomenon.72 There is an inverse correlation between BCL11A and HbF expression in erythroid cells. BCL11A occupies several discrete sites in the β-globin gene cluster and likely plays an important role in hemoglobin switching during erythroid differentiation.
Growth arrest-specific 6 (Gas6) protein is a secreted vitamin K–dependent protein that interacts with cell membranes and leads to intracellular signaling (via its receptor tyrosine kinases). Gas6 receptors are expressed in hematopoietic tissue, megakaryocytes, myelomonocytic precursors, and marrow stromal cells. Gas6 amplifies the erythropoietic response to EPO using a mouse model of Gas6 knockout.73 Gas6 is known to downregulate the expression of inflammatory cytokines such as tumor necrosis factor-α by macrophages.74
Figure 32-5 outlines the interrogation of the molecular mechanisms that regulate lineage-specific differentiation and commitment reveals the existence of separate megakaryocytic/erythroid progenitors versus both myeloid and lymphoid lineages.75
Figure 32–5.
Schematic outline of emerging hierarchy of hematopoiesis outlining a separate progenitor for erythroid/megakaryocytic lineage (MEP). RUNX-1, Scl1/Tal1, Lmo1, MllTel, PU-1, GATA-1, GATA-2, gf-1,C/EBPα, FOG1, EKLF, Ikaros, E2A, EBF, PAX-5, BCL 11A, NOTCH, TCF-1 are transcription factors. CLP, common lymphoid progenitor; CMP, common myeloid progenitor; GMP, granulocyte/macrophage progenitor; LT-HSC, long-term hematopoietic stem cell; MEP, megakaryocyte/erythroid progenitor; RBCs, red blood cells; ST-HSC, short-term hematopoietic stem cell. (Reproduced with permission from Orkin SH1, Zon LI: Hematopoiesis: an evolving paradigm for stem cell biology, Cell. 2008 Feb 22;132(4):631-644.)
The principal hormone regulating erythropoiesis is EPO, which is produced principally in the kidney.7 Erythroid progenitors express their own EPO.76 Different levels of kidney-produced EPO are optimal for various stages of erythroid maturation.77 Purification of EPO provided a partial protein sequence that led to cloning of the gene and permitted mass production of the recombinant protein.78 EPO and its recombinant form are heavily glycosylated α-globulins with a molecular mass of 34,000 daltons and a specific activity of approximately 200,000 IU/mg.79,80 Sixty percent of the molecular weight of the recombinant protein is contributed by amino acids; the remaining 40 percent is composed of carbohydrate. Using molecular probes for EPO, mRNA enabled the localization of the synthesis of EPO to renal cortical interstitial cells81,82 of endothelial or fibroblastic lineage. The cells appear to function in an all-or-none fashion, with the overall production of mRNA dependent on the number of cells activated.83
Certain 5′ sequences located 6000 to 12,000 bp upstream also affect EPO gene transcription.84 These sequences are not hypoxia sensitive but appear necessary for tissue and cellular specificity.84 Hepatic production is contributed primarily by hepatocytes but is a much less important source than is the kidney.85
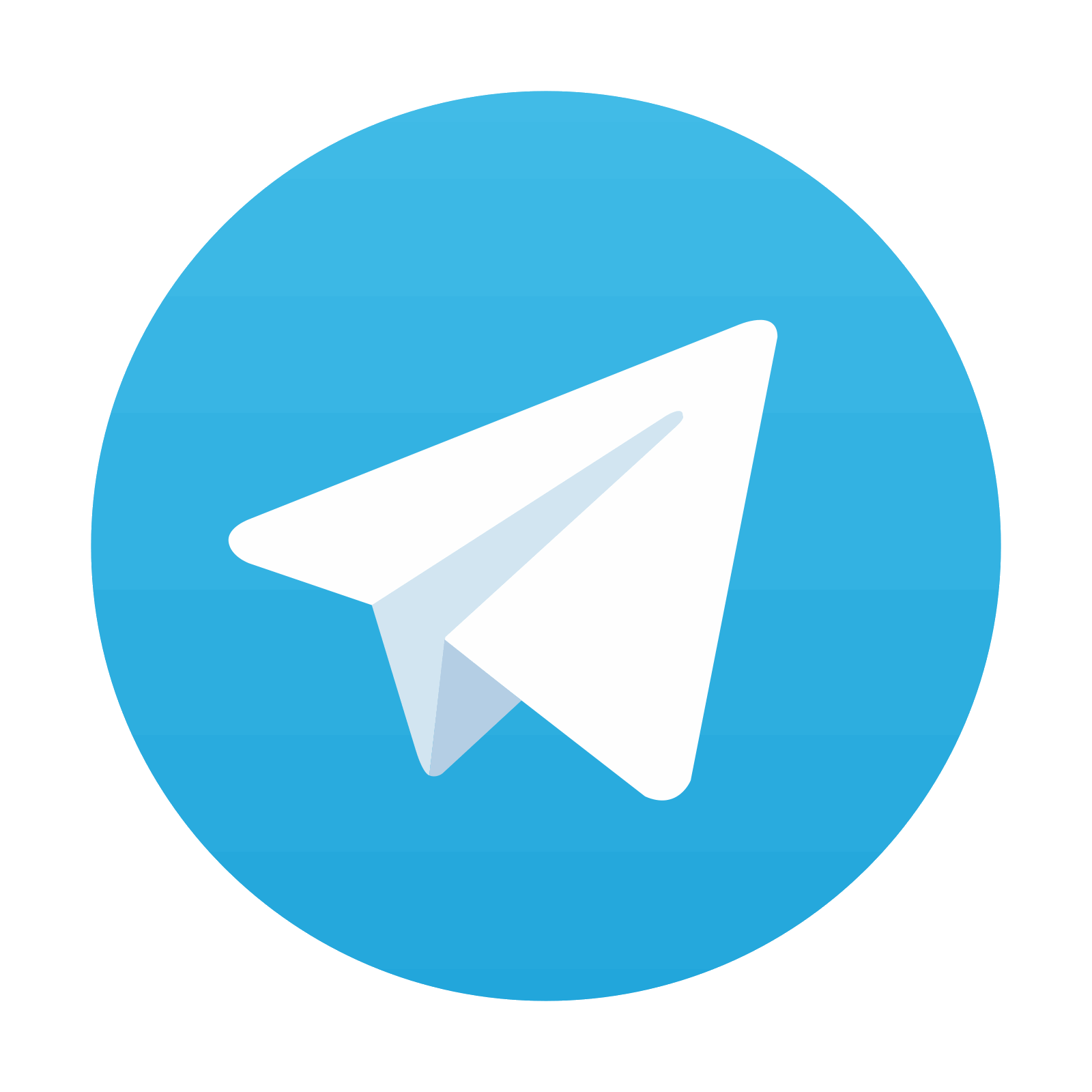
Stay updated, free articles. Join our Telegram channel
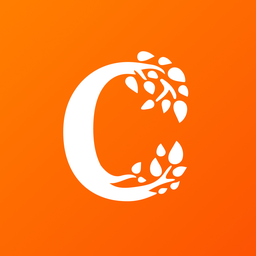
Full access? Get Clinical Tree
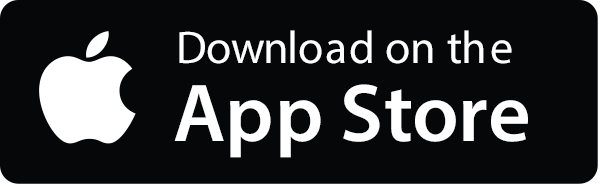
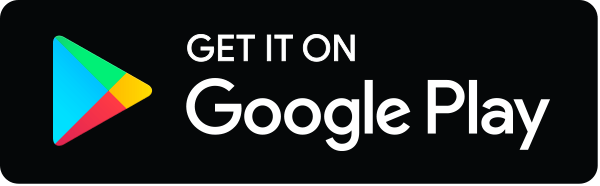
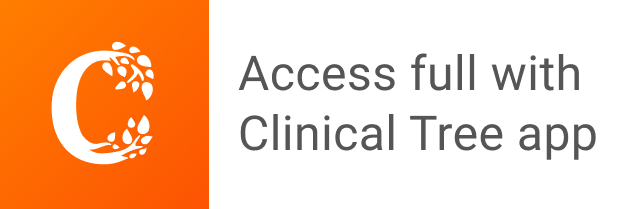