FIGURE 1 | Dopamine interactions in the CNS. Dopamine cells in the VTA express receptors for numerous molecules important in the central regulation of food intake. Dopamine neurons project widely to numerous areas that express D1 and D2 dopamine receptors. There are extensive interactions between these regions.
*Note: Receptor colocalization has not been experimentally examined for each receptor subtype.
Several lines of evidence, including data from animal models, genetic association studies, and human neuroimaging studies support the hypothesis of imbalanced dopamine availability and function in obesity. Rodent obesity models, either genetic or environmental, display changes in basal dopamine levels and altered levels of D2 receptor expression compared to lean animals (the direction and magnitude of which are dependent on brain region138–140) as well as differential dopamine response to feeding141–146 (e.g., both basal and ingestion-related DA release is increased in the LHA143). Similarly, genetic linkage studies in human populations have found a correlation between BMI categories and/or changes in food intake behaviors with polymorphisms of several dopaminergic genes including DAT (SLC6A4)147 dopamine receptors D2 and D4,147–155 as well as monoamine oxidase A.156 Given the extensive interactions between dopamine and other CNS neuropeptide systems important in the control of food intake and metabolism (e.g., NPY and melanocortins), dysregulation of the dopamine system could trigger a broad disruption of numerous facets of the CNS circuitry with significant implications for managing obesity. For example, a recent report identified a combination of alleles in two genes (DRD2 and mu-opioid receptor) that were overrepresented in obese individuals with binge-eating disorder (BED) compared to other obese patients without BED.157 Additionally, neuroimaging studies in obese subjects detected a decrease in dopamine D2 receptor 158 which correlated with reduced activity of PFC regions associated with inhibitory control and emotional reactivity.50,153 On the other hand, a number of studies have demonstrated that obese subjects have increased activation of brain regions corresponding to motivational and rewarding behaviors when exposed to food stimuli.38,50,159–161 Thus, it has been proposed that impaired inhibitory control over food intake, in addition to enhanced food reward and motivation for food consumption, may represent behavioral consequences of impaired dopaminergic circuitry in obesity.38,50,158,161
Finally, to better understand how repeated palatable food consumption may induce neuroadaptations in DA circuitry to perpetuate obesity, as well as how persistent DA imbalance can trigger appetitive changes, several groups have pointed to similarities between drug addiction and obesity.40,50,162,163 First, palatable food is a potent reinforcer with the ability to stimulate DA release in NAc. Additionally, food restriction and intermittent feeding increase dopamine release and therefore augment the rewarding effect of food,94,164 a characteristic of sensitization. On the other hand, repeated exposure to palatable food decreases dopamine activity in NAc which is a correlate of a habituation process.103,165,166 Chronic exposure to palatable diet also induces persistent changes in levels of available DA and alters expression of DA-related genes,167–172 indicating food-associated neuroadaptational changes in reward circuitry. Similarly, chronic consumption of sucrose can increase the locomotor response (sensitize) to amphetamine173 and cocaine,174 and increase the consumption of alcohol.175 Thus, neuronal mechanisms underlying addiction-like behavior after repeated food overconsumption and drug use are similar, and likely involve the dopaminergic reward pathway.50,103,161,176
POTENTIAL MOLECULAR MECHANISMS: PROGRAMMING OF DOPAMINERGIC CIRCUITRY
As mentioned earlier, gene–environment interactions are clearly important in understanding the etiology of obesity. It is evident that environmental insults (including exposure to suboptimal diet, toxic chemicals, or behavioral/stress conditions) occurring at vulnerable developmental time-points can induce phenotypic variations and possibly increase the risk of diseases, including obesity.177–179 During the prenatal and early postnatal time periods, organ systems are particularly vulnerable to environmental insults because of their rapid growth and development. Intrauterine and early life events have been shown to be important determinants of adult health and disease, with well-documented effects on metabolism,176,180–186 and specifically obesity (e.g., prenatal malnutrition,187 maternal obesity,188 smoking,186 stress,189 and immune activation190 have all been shown to increase the risk for obesity). On a molecular level such environmental manipulations can leave marks in gene regulatory regions (e.g., DNA methylation, histone acetylation and methylation) and lead to altered gene expression. Although such transient molecular changes are useful for quick adaptation of an organism to ever-changing environment, persistent epigenetic changes may lead to disease. Altered epigenetic status of various gene targets has been very well studied in cancer and some neurological diseases.191–193 Importantly, certain prenatal and early life manipulations have been shown to induce metabolic changes via epigenetic changes of various target gene systems.178,194–198
One potential underlying mechanism linking prenatal environment with postnatal obesity could be changes in the CNS circuitry that underlie food intake. Important effects of leptin on the development of hypothalamic appetite circuitry have been elegantly described.199 In a similar way, various environmental manipulations could induce molecular epigenetic changes in dopaminergic circuitry and induce reprogramming of neurobehavioral substrates related to food intake and food reward. The dopamine system appears sensitive to prenatal perturbations. A broad range of challenges have been examined and found to impact different aspects of the dopamine system, including gene expression of dopamine-related genes, dopamine levels in select brain regions, receptor function and expression, and dopamine-related behaviors. Consumption of a diet low in protein by a pregnant dam leads to low birth weight pups, a model for intrauterine growth retardation (which affects up to 10% of all babies born in the United States). Maternal low protein leads to alterations in dopamine in the offspring, including a decrease in basal levels of DA in the PFC as well as an absence of stress-induced release of DA.200 Exposure to a low protein diet from embryonic day 14 through 40 days postnatal led to a differential response to cocaine, including increased DA release in the NAc core and an increased sensitivity to cocaine.201,202 Pups that experienced protein malnutrition in utero in combination with a separation stress on postnatal days 2–8 demonstrated an increase in DA metabolism in the hypothalamus.203 In the situation of maternal overnutrition, consumption of a high-fat diet by a pregnant dam has also been demonstrated to affect the development of the DA system in the offspring. Consumption of a 30% fat diet from the last week of gestation through lactation resulted in increased TH mRNA in the VTA and NAc, and significant increases in dopamine in the NAc.204 Additionally, these offspring had a diminished locomotor response to amphetamine. A maternal diet that is deficient in the essential amino acid linolenic acid results in pups that have reduced levels of TH in the SN/VTA and increased expression of D1 and D2 receptors in the cortex and striatum.205 Similarly, offspring from iron deficient pregnancies had increased levels of TH and cellular dopamine in the striatum.206
In addition to diet, exposure to drugs or toxins during the prenatal period can also alter the development of the DA system. Maternal exposure to lead in the drinking water led to offspring with D2 receptor sensitivity and enhanced dopamine turnover in the striatum.207 Exposure of the pregnant dam to bisphenol-A resulted in pups with altered D1 and D2 functioning in the striatum208 and increased dopamine receptor function in the limbic forebrain.209 Exposure of the pregnant dam to inhaled diesel exhaust led to decreased DA turnover in the NAc and hypolocomotion.210 Prenatal exposure to cocaine can alter the expression and function of the D1 receptor,211,212 whereas fetal asphyxia at e17 led to decreased expression of TH, as well as hyperlocomotion, both basally and in response to amphetamine administration.213
Maternal stress can result in changes in the dopamine system of the developing offspring. Pups from mothers that experienced restraint stress during pregnancy showed a range of abnormalities, including increased D1 expression and DA turnover and decreased DAT and D2 expression in the striatum.214 In other studies using restraint, both basal and amphetamine-induced levels of DA were elevated in the NAc,215 and cocaine administration in the offspring led to an increase in DA release in the NAc and an exaggerated locomotor response.216 A potential mechanism could involve the release of endogenous glucocorticoids by the pregnant dam in response to the stressor. As predicted, administration of the synthetic glucocorticoid dexamethasone leads to an increase in TH in the SN and VTA.217
Finally, immune activation is also known to affect the development of the DA system. Pregnant dams injected with lipopolysaccharide (LPS; an immune system stimulant) gave birth to offspring that had reduced TH in the SN and reduced DA in the cortex, NAc, striatum, amygdala, and hypothalamus.218 Using a different immune activator (Poly I:C), mice were found to demonstrate an increase in TH expression in the caudate and NAc, as well as a hyperlocomotor response to cocaine injection. Interestingly, using a cross fostering design, this effect was also observed in animals from control pregnancies that were reared by mothers that received the Poly I:C during pregnancy, indicating an important postnatal maternal effect as well.
Collectively, these studies indicate that a broad range of environmental insults occurring in prenatal period, including suboptimal maternal diet, maternal stress or infection, and environmental toxins can alter the development of the dopamine system and have the potential to establish a predisposition for obesity, which in a permissive environment may lead to disease.
CONCLUSIONS
The importance of CNS mechanisms for the regulation of food intake and their relevance to ethiopathology of obesity are evident. Numerous homeostatic systems that maintain energy balance by engaging hypothalamic and brainstem mechanisms have been implicated in regulation of food intake. Recently, there has been accumulating evidence for the importance of hedonic circuits, including dopaminergic reward mechanisms, in regulation of food intake and the development of obesity. Furthermore, an indication for dopamine system dysfunction in obesity has been supported from human studies and in animal models. Depending on the specific brain region, time-point, and subtype of obesity under study, both ‘hypofunction’ and ‘hyperactivity’ of the dopaminergic system have been used to explain dopamine imbalance in obesity. On one hand, obesity-associated overindulgence may be a way to compensate (‘self-medicate’) for reduced dopamine levels. Conversely, an increased drive to consume palatable food may come as a consequence of overactive dopamine circuitry. This dichotomy has recently been thoroughly discussed.219 Furthermore, both genetic and epigenetic factors have a significant role in programming the response of neural reward/food intake circuits to obesogenic environment. With continued research, it should be possible to define the conditions under which dopamine dysfunction contributes to and/or results from obesity and related pathologies.
REFERENCES
1. Flegal KM, Carroll MD, Kuczmarski RJ, Johnson CL. Overweight and obesity in the United States: prevalence and trends, 1960–1994. Int J Obes Relat Metab Disord 1998, 22:39–47.
2. Ogden CL, Carroll MD, Curtin LR, McDowell MA, Tabak CJ, et al. Prevalence of overweight and obesity in the United States, 1999–2004. JAMA 2006, 295:1549–1555.
3. Ogden CL, Yanovski SZ, Carroll MD, Flegal KM. The epidemiology of obesity. Gastroenterology 2007, 132:2087–2102.
4. Ford ES, Mokdad AH. Epidemiology of obesity in the Western Hemisphere. J Clin Endocrinol Metab 2008, 93:S1–S8.
5. Ogden CL, Carroll MD, Flegal KM. High body mass index for age among US children and adolescents, 2003–2006. JAMA 2008, 299:2401–2405.
6. Lawrence VJ, Kopelman PG. Medical consequences of obesity. Clin Dermatol 2004, 22:296–302.
7. Kopelman PG. Obesity as a medical problem. Nature 2000, 404:635–643.
8. Flegal KM, Graubard BI, Williamson DF, Gail MH. Cause-specific excess deaths associated with underweight, overweight, and obesity. JAMA 2007, 298:2028–2037.
9. Kolotkin RL, Meter K, Williams GR. Quality of life and obesity. Obes Rev 2001, 2:219–229.
10. Muennig P. The body politic: the relationship between stigma and obesity-associated disease. BMC Public Health 2008, 8:128.
11. Sarlio-Lahteenkorva S, Stunkard A, Rissanen A. Psychosocial factors and quality of life in obesity. Int J Obes Relat Metab Disord 1995, 19(suppl 6):S1–S5.
12. Finkelstein EA, Fiebelkorn IC, Wang G. National medical spending attributable to overweight and obesity: how much, and who’s paying? Health Aff (Millwood) 2003. (Suppl Web Exclusives:W3:219–226).
13. Sturm R. The effects of obesity, smoking, and drinking on medical problems and costs. Health Aff (Millwood) 2002, 21:245–253.
14. Thorpe KE, Florence CS, Howard DH, Joski P. The impact of obesity on rising medical spending. Health Aff (Millwood) 2004. (Suppl Web Exclusives:W4:480–486).
15. Finkelstein EA, Trogdon JG, Brown DS, Allaire BT, Dellea PS, et al. The lifetime medical cost burden of overweight and obesity: implications for obesity prevention. Obesity (Silver Spring) 2008, 16:1843–1848.
16. Trogdon JG, Finkelstein EA, Hylands T, Dellea PS, Kamal-Bahl SJ. Indirect costs of obesity: a review of the current literature. Obes Rev 2008, 9:489–500.
17. Finkelstein EA, Trogdon JG, Cohen JW, Dietz W. Annual medical spending attributable to obesity: payer- and service-specific estimates. Health Aff (Millwood) 2009, W8:22–83.
18. Thorleifsson G, Walters GB, Gudbjartsson DF, Steinthorsdottir V, Sulem P, et al. Genome-wide association yields new sequence variants at seven loci that associate with measures of obesity. Nat Genet 2009, 41:18–24.
19. Willer CJ, Speliotes EK, Loos RJ, Li S, Lindgren CM, et al., Genetic Investigation of ANthropometric Traits Consortium. Six new loci associated with body mass index highlight a neuronal influence on body weight regulation. Nat Genet 2009, 41:25–34.
20. Farooqi IS, O’Rahilly S. Monogenic human obesity syndromes. Recent Prog Horm Res 2004, 59:409–424.
21. Chung WK, Leibel RL. Considerations regarding the genetics of obesity. Obesity (Silver Spring) 2008, 16(suppl 3):S33–S39.
22. August GP, Caprio S, Fennoy I, Freemark M, Kaufman FR, et al. Endocrine Society: prevention and treatment of pediatric obesity: an endocrine society clinical practice guideline based on expert opinion. J Clin Endocrinol Metab 2008, 93:4576–4599.
23. Cope MB, Allison DB, Critical review of the World Health Organization’s (WHO). 2007 report on ‘evidence of the long-term effects of breastfeeding: systematic reviews and meta-analysis’ with respect to obesity. Obes Rev 2008, 9:594–605.
24. Qi L, Cho YA. Gene-environment interaction and obesity. Nutr Rev 2008, 66:684–694.
25. Marti A, Martinez-Gonzalez MA, Martinez JA. Interaction between genes and lifestyle factors on obesity. Proc Nutr Soc 2008, 67:1–8.
26. Levin BE. Epigenetic influences on food intake and physical activity level: review of animal studies. Obesity (Silver Spring) 2008, 16(suppl 3):S51–S54.
27. Abizaid A, Horvath TL. Brain circuits regulating energy homeostasis. Regul Pept 2008, 149:3–10.
28. Berthoud HR, Morrison C. The brain, appetite, and obesity. Annu Rev Psychol 2008, 59:55–92.
29. Lenard NR, Berthoud HR. Central and peripheral regulation of food intake and physical activity: pathways and genes. Obesity (Silver Spring) 2008, 16(suppl 3):S11–S22.
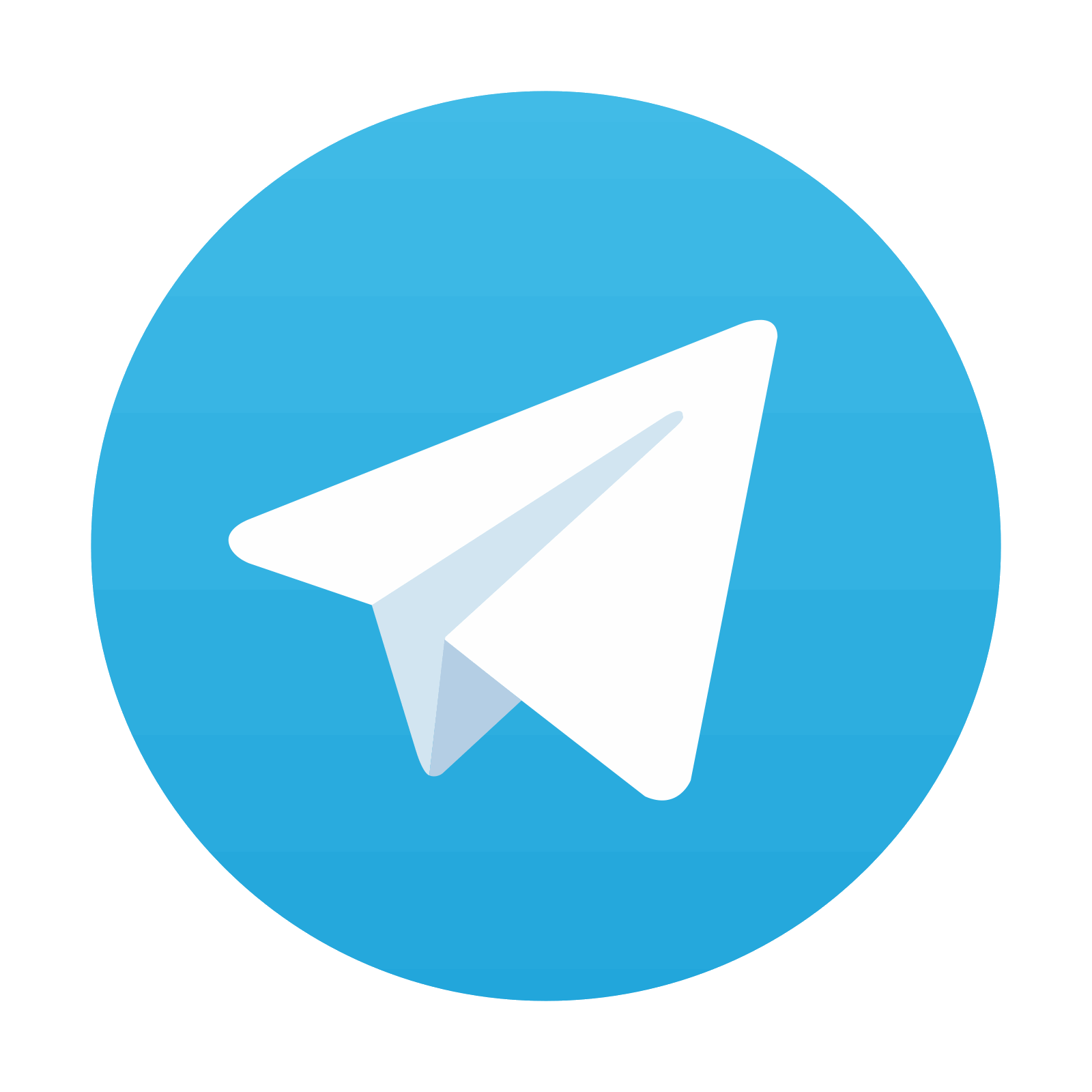
Stay updated, free articles. Join our Telegram channel
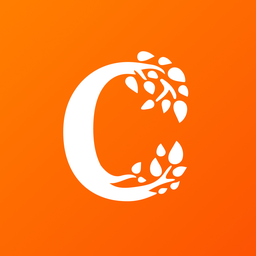
Full access? Get Clinical Tree
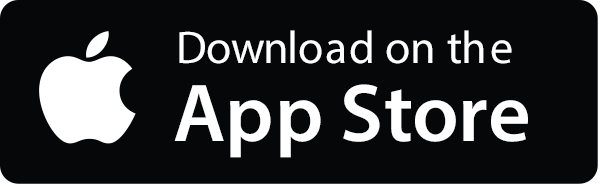
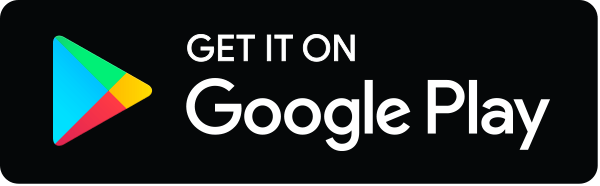