CEREBROSPINAL FLUID IN CENTRAL NERVOUS SYSTEM INFECTIONS
RODRIGO HASBUN
Infections within the central nervous system (CNS) frequently, but not always, produce changes in cerebrospinal fluid (CSF). The changes produced may provide invaluable information about the nature of the infectious process and, in many cases, may permit specific identification of the offending organism. Despite the great diagnostic value of CSF analysis, however, injudicious attempts to obtain CSF (as in the setting of increased intracranial pressure) can sometimes cause brain herniation or death, and casual handling of the CSF obtained may render the analysis useless.
This chapter is divided into three parts. The first part reviews the anatomy of the CSF spaces, the physiology of CSF production and reabsorption, and the effect of infection on CSF physiology and composition. The second part discusses methods of CSF analysis in CNS infections, and the third part summarizes the CSF analysis in specific CNS infections.
ANATOMY AND PHYSIOLOGY OF THE CEREBROSPINAL FLUID COMPARTMENTS
The CSF is contained within two connecting compartments, the cerebral ventricles and the subarachnoid space (1). Infectious organisms may affect both compartments, and analysis of CSF from both may reflect changes produced by infectious or parainfectious processes within meninges, brain, or spinal cord.
The Ventricular System
The cerebral ventricular system represents, in greatly elaborated form, the remnants of the embryologic neural tube. A single layer of neuroglial-derived cells, the ventricular ependyma, lines the ventricles; a dense network of astrocytic foot processes backs these. The ventricular system consists of two lateral ventricles, the third ventricle, and the fourth ventricle (Fig. 2.1). The lateral ventricles are located within the cerebrum and consist of frontal, temporal, and occipital horns; these join at the ventricular trigone within the parietal lobe. The third ventricle is an elongated, slitlike cavity that lies within the midbrain and is bounded inferiorly by the hypothalamus. The fourth ventricle overlies the brainstem from the level of the midpons to the extreme rostral end of the spinal cord. The roof of the fourth ventricle is the cerebellum posteriorly and the superior and inferior medullary veli anteriorly. The fourth ventricle is roughly diamond shaped and is widest at the lateral recesses, which lie between the superior and middle cerebral peduncles.
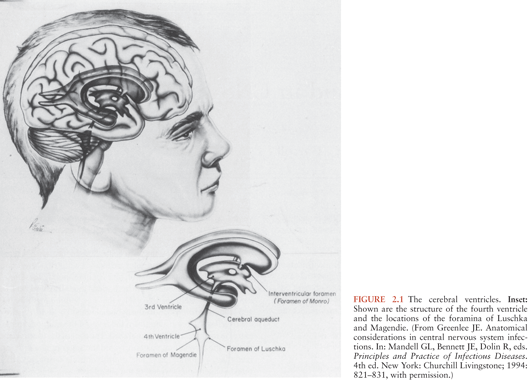
The cerebral ventricles are connected to each other and with the subarachnoid space through a series of small openings. Each lateral ventricle drains into the third ventricle through the foramen of Monro, located in the inferomedial wall of the frontal horn. The third and fourth ventricles are connected by the aqueduct of Sylvius, which extends through the midbrain. The fourth ventricle drains into the subarachnoid space through three small openings, the foramina of Luschka and the foramen of Magendie. The foramina of Luschka are located in the lateral recesses of the fourth ventricle and are absent in up to 20% of the population. The foramen of Magendie is located in the midline and, in most persons, represents the major communication between the fourth ventricle and the subarachnoid space. As is discussed later, these narrow openings are important in CNS infections because they represent the sites at which obstruction of CSF flow may most easily occur.
The Meninges and Subarachnoid Space
The brain and spinal cord are surrounded by three layers of meninges (2). The outermost layer of the meninges is a tough fibrous membrane, the dura mater. Within the skull, the dura forms the inner layer of the cranial periosteum and is tightly adherent to bone. Below the foramen magnum, the dura and periosteum diverge and are separated by a fat-filled epidural space. The middle layer of meninges, the arachnoid, is joined to the dura by a specialized layer of fibroblasts, the dural border cell layer. The cells of this inner dural border are devoid of collagen and have few cellular junctions, providing a cleavage plane in which infection may develop and rapidly spread. The arachnoid covers the brain and spinal cord loosely and extends outward along the course of cranial and spinal nerves.
The third layer of meninges, the pia mater, is continuous with the surface of the brain and spinal cord. The pia mater also follows vessels into brain and spinal cord parenchyma and projects into the ventricles to form the choroid plexuses. The pia mater and the ventricular ependyma merge at the foramina of Luschka and Magendie. The CSF is contained in the subarachnoid space, enclosed between the arachnoid and the pia. The subarachnoid space surrounds the brain and extends within the spinal canal to the level of the second sacral vertebra. Within the skull, the subarachnoid space widens into cisterns where pia and arachnoid are more widely separated by irregularities in the contour of the brain. The largest of these, the cisterna magna, surrounds the brainstem and the cerebellum at the base of the skull and is occasionally used as a source of CSF for analysis and culture. The subarachnoid space is crossed by trabecular extensions of the arachnoid itself, by cranial nerves, by a network of small arteries, the rete mirabile, and by numerous bridging veins, which connect the meningeal veins with the deeper intracranial venous system (2).
The subarachnoid space is normally a closed system. Occasionally, however, congenital or posttraumatic communications may exist between the subarachnoid space and superficial tissues and may provide a route for single or recurrent episodes of meningitis. Congenital defects arise from incomplete closure of the neural tube. These defects may extend for variable distances into subcutaneous tissues or to the cutaneous surface and are most common in the upper cervical regions and over the sacrum. Their presence may be suggested by a cutaneous dimple or a patch of hair. Traumatic communications into the subarachnoid space are most often associated with basilar skull fractures. The most common sites of involvement are (a) the thin layers of bone that separate the cranial cavity from the paranasal sinuses and (b) the petrous bone, which separates the auditory canals and mastoid from the cranial cavity. In rare instances, traumatic defects may occur over the cranial convexities or along the spinal column.
PHYSIOLOGY OF CEREBROSPINAL FLUID PRODUCTION AND REABSORPTION
CSF is produced by the choroid plexuses of the lateral, third, and fourth ventricles and, to a lesser extent, by extrachoroidal sites (1,3). In adults, the choroid plexus produces approximately 500 mL of CSF per day, with 150 mL present in the ventricular system at any time. The choroid plexuses are specialized projections of vessels and pia mater into the ventricular cavities. Each choroid plexus branches into frondlike villi, each of which contains a capillary surrounded by loose connective tissue and a layer of specialized ependymal cells termed choroid epithelium. Choroidal epithelial cells, in contrast to ependymal cells elsewhere in the ventricular system, are columnar in shape and are covered on their ventricular surfaces by a brush border of microvilli. The villous structure of the choroid plexus and the presence of microvilli greatly increase the surface area available for secretion of CSF (1).
Formation of CSF involves both filtration and active transport (1,3). Filtration of CSF varies inversely with serum osmolality. In experimental animals, and possibly in humans, CSF production changes 7% for each 1% change in serum osmolality (4). Active secretion of CSF involves Na+, K+-adenosine triphosphatase (ATPase)–mediated transport of sodium across choroidal epithelium into the ventricular lumen, with water, chloride, and bicarbonate ions following through facilitated transport. In experimental animals, the carbonic anhydrase inhibitor acetazolamide reduces CSF secretion by approximately 50%, whereas furosemide and ethacrynic acid reduce CSF production by 25% to 35% (5). Simultaneous use of both agents reduces CSF formation by 75%.
Reabsorption of CSF occurs through arachnoid villi. Most of these are located along the superior sagittal sinus. Smaller numbers of arachnoid villi are found along other intracranial venous sinuses and around spinal nerve roots (1). During health, the arachnoid villi along the superior sagittal sinus provide the major site of CSF uptake. The arachnoid villi along other sinuses and surrounding spinal nerve roots may provide alternative sites of CSF absorption following superior sagittal sinus thrombosis.
Each arachnoid villus represents an extension of the arachnoid membrane through the dura mater into the lumen of the venous sinus and functions as a one-way valve, permitting unidirectional flow from CSF into blood. Early work by Welch (6) demonstrated that the arachnoid villi have a critical in vitro opening pressure of 2 to 5 cm H2O; this study also demonstrated that particles up to the size of erythrocytes readily pass from CSF into blood, whereas particles larger than 7.5 µm are excluded. Although these early data suggested that the arachnoid villi might provide a direct communication between CSF and blood, studies using electron microscopy have demonstrated that arachnoid villi and venous sinuses are separated by a layer of endothelial cells connected by tight junctions, and that movement of CSF and particulate matter across the arachnoid villi occurs by transport within giant vesicles (7,8) (Fig. 2.2). These giant vesicles, although they provide efficient transfer of CSF into blood under normal circumstances, can become obstructed by bacteria and inflammatory cells during meningitis or by red blood cells (RBCs) during subarachnoid hemorrhage (9,10).
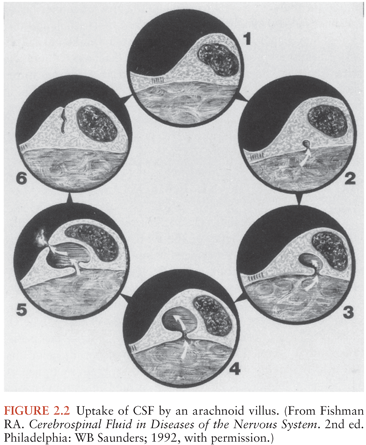
BRAIN AND CEREBROSPINAL FLUID BARRIER SYSTEMS
The brain and CSF are contained within a series of barrier systems (1). These prevent entry of fluids, electrolytes, and other substances from blood into CSF or brain by simple diffusion and isolate the CNS from systemic immune responses. The blood–brain barrier (BBB) is formed by tight junctions between endothelial cells of CNS capillaries and is further reinforced by a surrounding layer of astrocytes, whose processes terminate in overlapping fashion on the capillary walls. In contrast, the blood–CSF barrier is formed by the endothelial cells of the choroid plexus and the tight junctions that link them. The cells of the pia mater, like those of choroid plexus and arachnoid capillaries, are separated by gap junctions; entry of substances from CSF into brain is modulated by a basement membrane subjacent to the pia and by a continuous layer of astrocytes beneath the basement membrane, forming a CSF–brain barrier.
The barrier systems that surround spinal cord and brain exclude from the CNS most of the immunologic mechanisms that provide host defense elsewhere in the body. Normally, T cells and B cells are present in very small numbers in CSF and only rarely in brain; immunoglobulins and complement are largely excluded from both CSF and brain; and opsonic activity of CSF, even in the presence of meningitis, is far less than that of serum (11–14). Therefore, both the brain and the CSF are poorly equipped to deal with infectious agents.
The barrier systems that isolate CSF, brain, and spinal cord from blood are not static systems but, instead, are highly dynamic in their ability to interact with and transport a wide variety of substances (15). In addition, it is increasingly recognized that the endothelial cells and astrocytes of the BBB and the blood–CSF barrier are important sources of cytokines (including tumor necrosis factor [TNF] and interleukins), and that astrocytes, in addition to their abilities to regulate solute entry into brain, have the ability to act as antigen-presenting cells (16). The release of cytokines by endothelial cells and astrocytes in response to bacterial endotoxins and other bacterial products is fundamental in the production of inflammation and injury during CNS infections and provides an extremely important area for early therapy (9,17–19).
MAINTENANCE OF CEREBROSPINAL FLUID HOMEOSTASIS
The BBB and the blood–CSF barrier maintain the cellular and chemical elements of the CSF within narrow ranges (1,3,20). Lipid-soluble substances within blood readily diffuse across choroidal epithelium or vascular endothelium into CSF or brain (3). Passage of fluid and ionically polar substances, however, requires mechanisms for transport and facilitated diffusion. Sodium enters CSF both by Na+, K+-ATPase–mediated transport during secretion of CSF and by passive diffusion (20). Potassium is secreted into CSF by active transport mechanisms and is actively removed from CSF into brain by transport mechanisms that are believed to be located in astrocyte foot processes. Movement of calcium, magnesium, and phosphorus into CSF and brain also occurs predominantly by active transport, and the concentrations of these substances are relatively independent of their concentrations in serum. Chloride and bicarbonate, like potassium, are actively secreted into and actively removed from CSF. Glucose, amino acids, amines, and thyroid hormone enter the brain by carrier-mediated transport mechanisms (1,15). Insulin and transferrin require receptor-mediated transport (15). Although lipids complexed to proteins were once thought to be excluded from the CNS, it is now known that complexed lipids undergo dissociation from their carrier proteins at the blood–brain interface and may enter the CNS without significant exodus of protein from brain capillaries (15).
Chloride represents the major anion in CSF. Normal CSF chloride concentration is 15 to 20 mEq/L higher than that in serum. Early workers observed that CSF chloride concentrations were lowered in tuberculous meningitis; for many years, levels of CSF chloride were used to diagnose and follow the course of this infection (1). It is now recognized, however, that the lowered CSF chloride concentration observed in tuberculous meningitis is nothing more than a reflection of lowered serum chloride values and has no diagnostic or prognostic value.
The acid–base balance of the CSF, like its electrolyte concentration, tends to remain fairly constant despite fluctuations in systemic acid–base balance. In CSF, as opposed to plasma, however, movement of CO2 occurs readily by diffusion, whereas movement of bicarbonate occurs more slowly by carrier-mediated transport. The discrepancy in the rate of movement of these two substances may produce delayed (and, at times, paradoxical) responses in CSF pH as compared to systemic pH during rapid changes in bicarbonate concentration (1). The CSF acid–base balance is also maintained by the choroid plexuses, which possess transport mechanisms capable of removing weak organic acids—including antibiotics such as the penicillins, cephalosporins, and aminoglycosides—from CSF (21,22). Choroid plexus transport of antibiotics and other weak organic acids can be blocked by probenecid.
ALTERATIONS OF CEREBROSPINAL FLUID DYNAMICS AND PRESSURE IN CENTRAL NERVOUS SYSTEM INFECTIONS: HYDROCEPHALUS, INTRACRANIAL HYPERTENSION, AND BRAIN HERNIATION
Acute or chronic CNS infections may produce profound alterations in intracranial pressure (ICP) by obstructing CSF flow or reabsorption, by behaving as space-occupying lesions, or by producing hemorrhage or cerebral edema. These pathologic consequences of infection, acting individually or together, may cause brain herniation and death.
Alteration of Cerebrospinal Fluid Circulation in Central Nervous System Infections
Impairment of normal CSF circulation may result in ventricular enlargement and hydrocephalus. Interruption of CSF reabsorption produces communicating hydrocephalus with normal circulation of CSF through the ventricular system and into the subarachnoid space. Communicating hydrocephalus is a common complication of bacterial meningitis and, in most cases, results from obstruction of the arachnoid villi by bacteria and white blood cells (WBCs) (9). Communicating hydrocephalus may also result from functional occlusion of arachnoid villi during severe meningitis or by RBCs in the course of subarachnoid hemorrhage during bland or septic subarachnoid hemorrhage (10). Thrombosis of the superior sagittal sinus may also block CSF reabsorption and thereby produce communicating hydrocephalus. Occlusion of a large portion of the superior sagittal sinus usually produces catastrophic, often hemorrhagic, cerebral infarction. Involvement of the anterior third of the sinus, however, may be clinically silent except for the development of hydrocephalus.
Obstructive hydrocephalus results from interruption of CSF flow within the ventricular system or at its point of exit into the subarachnoid space (2). This may be the consequence of infection of the ventricular ependyma or basilar meninges or may result from extrinsic compression of the ventricular system by infection within brain parenchyma. Lesions producing obstructive hydrocephalus most commonly involve the ventricular system at its narrowest points: the foramina of Luschka and Magendie, the fourth ventricle, the aqueduct of Sylvius, and the foramina of Monro. Obstruction of the foramina of Luschka and Magendie is characteristic of exudative basilar meningitides such as those caused by Mycobacterium tuberculosis, Coccidioides immitis, and Cryptococcus neoformans but may also be seen in bacterial meningitis. Hydrocephalus as a result of obliteration of the fourth ventricle is almost always extrinsic and is the result of ventricular compression by large cerebellar mass lesions such as cerebellar abscess or hemorrhage. Occlusion of the aqueduct of Sylvius by granulomatous ependymitis may occur as a complication of tuberculosis, fungal infections, or sarcoidosis. Mumps virus, which replicates in ventricular ependymal cells, has been shown to produce congenital aqueductal stenosis in experimental animals (23). Rare cases of hydrocephalus have also been reported following mumps and with Toscana meningoencephalitis in humans (24,25). Extrinsic compression of the aqueduct of Sylvius may be produced by abscesses or other localized infections within the pons or midbrain. Involvement of the foramen of Monro is almost always unilateral and is the consequence of severe brain shifts caused by abscess, focal encephalitis, or hemorrhage. Hydrocephalus caused by the occlusion of one foramen of Monro is particularly dangerous because the CSF trapped within the involved lateral ventricle acts as a unilateral space-occupying lesion, greatly increasing the risk of transtentorial brain herniation.
Computerized tomography (CT) and magnetic resonance imaging (MRI) are invaluable in demonstrating the presence of hydrocephalus and in determining its cause. Ventricular dilation is common in the elderly and is characterized by symmetric ventricular dilation accompanied by evidence of cerebral cortical atrophy. In contrast, hydrocephalus is defined as a frontal horn ratio (Evans index) of 0.3 or greater in the absence of cerebral atrophy (26). Hydrocephalus that occurs from impaired CSF circulation is accompanied by loss of cortical markings visible on CT or MRI as the brain is forced outward against the skull and by periventricular areas of increased lucency, representing transependymal leakage of CSF. Communicating hydrocephalus and hydrocephalus from obstruction of the foramina of Luschka and Magendie are characterized by symmetric enlargement of all four ventricles. Hydrocephalus from occlusion of the fourth ventricle or aqueduct of Sylvius results in loss of that structure on CT or MRI, with dilation of the third and lateral ventricles. Hydrocephalus following compression of the foramen of Monro is almost invariably associated with an identifiable space-occupying lesion and a prominent midline shift. Thrombosis of the superior sagittal sinus may be difficult to detect as a cause of communicating hydrocephalus and can be missed with the use of routine CT scanning. MRI and CT venogram are more sensitive and are used to diagnose superior sagittal sinus (SSS) thrombosis (27).
Intracranial Hypertension and Brain Herniation
The normal mechanisms of CSF secretion and drainage maintain CSF pressure at a level less than 150 mm of CSF in most patients. Infection, however, greatly alters these homeostatic mechanisms; moreover, death during the acute stages of intracranial CNS infections often results from extreme elevation in ICP followed by brain herniation and respiratory arrest.
For a period of time, the intracranial contents are able to compensate in response to space-occupying lesions before a rise in ICP occurs. This compensatory ability is termed compliance (dV/dP) and represents the ratio of changes in volume (dV) to changes in pressure (dP). Compliance in response to space-occupying intracranial lesions consists of several factors. These include increased rate of reabsorption of CSF (this may be prevented in meningitis by obstruction of the arachnoid villi by cells and exudate); displacement of CSF; reduction in the total volume of intracranial blood, predominantly by compression of veins and venous sinuses; and plasticity of the brain itself. Compliance is extremely limited when infection is accompanied by a rapid increase in ICP, such as during acute bacterial meningitis or subdural empyema. In contrast, the ability of CNS compliance to compensate for increased ICP may be extensive where space-occupying lesions develop over time (28). Once compliance is exceeded, however, the increase in pressure in chronic lesions may occur rapidly.
The elevation in CSF pressure seen in infections and other pathologic conditions is not constant but fluctuates considerably. This fluctuation is usually not observed during the brief period of measurement provided by LP but becomes an important parameter to observe during monitoring of ICP. Minor variation in pressure occurs during Cheyne-Stokes respiration and during variations in blood pressure produced by Hering-Breuer reflexes, the inflation and deflation reflexes that help regulate the rhythmic ventilation of the lungs. More major variations in ICP occur during plateau waves. These are abrupt elevations in ICP (usually lasting 5 to 20 minutes) in which ICP may reach 600 to 1,300 mm of CSF (50 to 100 mm Hg) (29,30). Plateau waves are believed to represent a consequence of disturbed cerebrovascular autoregulation because of either abnormal sympathetic tone or cyclic changes in perfusion in which mild hypotension is followed by cerebral vasodilation and increased cerebral blood flow (30). Although plateau waves may be without any detectable clinical effect, they may also be associated with signs of brainstem compression and impending herniation.
Increased pressure that exceeds intracranial compliance causes downward and backward shifting of the cerebrum and brainstem (31). Minimal degrees of shift are well tolerated, but a more extensive shift may cause herniation of the cingulate gyrus beneath the falx cerebri, herniation of the uncus of the temporal lobe over the tentorium cerebelli, and ultimately, herniation of the lower brainstem and cerebellar tonsils into the foramen magnum. Herniation of the cingulate gyrus is usually asymptomatic. Uncal herniation, however, initially produces compression of the third cranial nerve as it passes beneath the tentorium; it subsequently causes compression of the midbrain, with resultant coma. The aqueduct of Sylvius is often occluded during uncal herniation, and the resultant hydrocephalus increases the mass effect already present. Herniation of the cerebellar tonsils through the foramen magnum, with compression of medullary respiratory centers and respiratory arrest, is often the terminal event in CNS infections. Occasionally, space-occupying lesions within the cerebellum cause upward herniation of posterior fossa contents through the tentorial notch (32). Extreme elevation of CSF pressure may elevate ICP above systemic arterial perfusion pressure, producing global cerebral and brainstem infarction.
Elevation in CSF pressure, as monitored by ICP monitoring devices, may provide an indication of prognosis in bacterial meningitis and possibly in other CNS infections. Rebaud et al. (33) found that CSF pressures were significantly higher and cerebral perfusion pressure were significantly lower (mean systemic arterial pressure minus ICP) in patients who died due to meningitis or encephalitis than in those who survived. Goitein and Tamir (34) found that all pediatric patients with meningitis or encephalitis who had a cerebral perfusion pressure more than 30 mm Hg survived, whereas those with lower pressures died.
CEREBROSPINAL FLUID ANALYSIS IN CENTRAL NERVOUS SYSTEM INFECTIONS
Indications for Lumbar Puncture
LP is essential in the diagnosis of bacterial, viral, or fungal meningitis and may provide valuable information in encephalitis. LP is also used to diagnose subarachnoid hemorrhage in patients with a negative head CT scan. The procedure is of little specific diagnostic value in the diagnosis of brain abscess or parameningeal infections. Lumbar punctures (LPs) should not be done in patients with impending herniation or with intracranial mass lesions with severe mass effect. Furthermore, inappropriate LP can cause patient death or serious neurologic injury, and the procedure should never be initiated without consideration of its potential danger to the patient.
Clinicians have relied on the meningeal signs (nuchal rigidity, Kernig sign, Brudzinski sign) for over 100 years to evaluate patients with suspected meningitis to help them decide who should undergo a LP. A prospective study of 297 adults with suspected meningitis documented a very low sensitivity of the Kernig sign (sensitivity, 5%), Brudzinski sign (sensitivity, 5%), and nuchal rigidity (sensitivity, 30%) (35). The absence of the meningeal signs should not defer the performance of the LP. The decision to perform a LP on those suspected of having meningitis is largely based on a combination of clinical signs and symptoms at presentations. The classic triad of fever, stiff neck, and altered mental status was present in only 44% of patients in a prospective study involving 696 patients with confirmed bacterial meningitis (36). However, at least two of the four symptoms of headache, fever, neck stiffness, and altered mental status were found in 95% of patients.
Major Complications of Lumbar Puncture
Role of Head Computerized Tomography Scan Before Lumbar Puncture and Risk of Brain Herniation
It has become a routine practice to obtain a CT scan of the head prior to performing a LP in patients with suspected meningitis. This is done to “rule out” the possibility of an intracranial mass, hydrocephalus, edema, or any other signs of increased ICP that could theoretically place the patient at risk for cerebral herniation after CSF removal during the LP (37). Herniation of the brain as the consequence of severe cerebral edema or acute hydrocephalus can sometimes occur in acute bacterial meningitis and other CNS infections. Clinically, this is manifested by altered state of consciousness, abnormalities in pupil reflexes, and decerebrate or decorticate posturing. The incidence of herniation after LP even in patients with papilledema is approximately 1% (37).
In order to clarify the role of a screening CT scan, a prospective study involving 301 adults with suspected meningitis was done (38). Baseline characteristics that were associated with an abnormal finding on head CT were age 60 years and older, immunocompromised host (i.e., HIV/AIDS, immunosuppressive therapy, or transplantation), a history of CNS disease, a history of seizure within 1 week before presentation, and any abnormality on neurologic examination. These factors have now been included in the Infectious Diseases Society of America guidelines to decide who should undergo CT prior to the LP (39). The decision to obtain a brain CT scan before LP should not result in delay in instituting antibiotic therapy because delay can increase mortality (40). It should be also noted that herniation can occur in patients with bacterial meningitis who have a normal brain CT scan. The most reliable clinical signs of “impending” herniation include deteriorating level of consciousness, brainstem signs, and a very recent seizure (41).
Spinal Hematoma with Cord Compression
Case reports of LP in patients with severe disorders of blood coagulation, thrombocytopenia, or in patients anticoagulated with heparin or Coumadin have described complications with either continued bleeding at the site of puncture or with epidural or subdural hematomas that may compress the cauda equina, thereby producing permanent neurologic injury (42). These complications appear to be rare. In a study of 5,223 LPs performed, no complications were seen in 941 children with leukemia who had severe thrombocytopenia (platelet count <50) (43).
Introduction of Infection into the Subarachnoid Space
Inadvertent LP through an area of infection overlying the spinal canal may result in seeding of the subarachnoid space and meningitis. This is a particular risk in spinal epidural abscess or subdural empyema but may occasionally occur in the setting of superficial or deep paraspinal infections. The problem can be avoided by entering the subarachnoid space at a level well removed from the site of presumed infection. Thus, in patients with known or suspected focal lumbar infection, spinal fluid should be obtained under fluoroscopic guidance by high cervical (C2) or cisternal puncture, whereas the lumbar route should be used in patients with suspected cervical or upper thoracic infections. Introduction of infection into the subarachnoid space during LP in uninfected individuals has been reported in 1 out of 50,000 LPs (44). The most commonly implicated organism is Streptococcus salivarius, and this could be potentially prevented by using a mask during the procedure (45).
Post–Lumbar Puncture Headache
The most frequent complication of LP is the post–LP headache, which can occur in 10% to 60% of patients, more commonly in young women with a lower body mass index (BMI) and in pregnancy (46). The diagnosis is a clinical one, and it is usually defined as a bilateral headache that worsens while sitting up and improves lying down, develops within 7 days after a LP, and disappears within 14 days (Fig. 2.3). It is thought that the headache is caused by a CSF leak that decreases ICP. This causes headache either by gravitational traction on sensitive meningeal vascular coverings as a result of CSF volume depletion or by activation of adenosine receptors as a result of decreased CSF volume, which would cause cerebral vasodilatation and stretching of pain-sensitive cerebral structures (46).
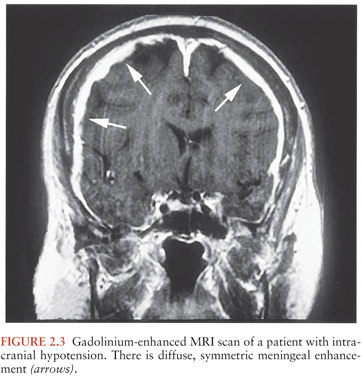
The incidence of the post–LP headache is not associated with the volume of CSF removed, hydration, the position of the patient (lying on their side or sitting up), or the opening pressure (47). Factors that can be associated with a decrease in the incidence of headache are the type and size of needle, the direction of the bevel during needle placement, the replacement of the stylet, and possibly the number of LP attempts (47). Atraumatic needles with a blunt end are recommended by the American Academy of Neurology to reduce the incidence of post–LP headaches (48). The “blunt” end produces a more traumatic opening with tearing and disruption of the collagen fibers that is closed faster by an immunologic reaction and thus associated with a decrease incidence of headache (49). Additionally, smaller needles have been shown to decrease the incidence of the post–LP headache (46). The direction of the bevel should be parallel to the long axis of the spine to decrease the incidence of headache. If the patient is lying on his or her side, the bevel should face “up.” This way, the needle will separate the dural collagen fibers, which also run along the long axis of the spine, rather than cutting them (47).
Several techniques to treat the post–LP headache exist including the instillation of a “blood patch,” dextran, or saline into the epidural space. A “blood patch” refers to the injection of 20 to 30 mL of the patient’s fresh blood into the epidural space. It is thought to work by closing the CSF leak by forming a clot, and it works in about 70% to 98% of patients (47). If a blood patch does not work, 20 mL of dextran or saline can be injected into the epidural space to raise the epidural pressure and reduce the CSF leak. Oral or intravenous caffeine can be used because they act as a cerebral vasoconstrictor and blocks adenosine receptors. Surgical closure of the dural gap is the last resort (46).
Less Common Complications of Lumbar Puncture
Cortical Blindness. Downward displacement of the brainstem in states of increased ICP may compress the posterior cerebral arteries against the edge of the tentorium cerebelli, causing ischemic infarction of the occipital lobes and cortical blindness (31). Although this complication of intracranial hypertension is often accompanied by signs of uncal or tonsillar herniation, compression of the posterior cerebral arteries may also occur before other signs of herniation appear. Prognosis for return of vision is poor.
Cervical Spinal Cord Infarction. Rarely, LP in the setting of bacterial meningitis may be followed within a few hours by respiratory arrest accompanied by flaccid tetraplegia (50). A variety of mechanisms, including hypotension and vasculitis, have been postulated as the cause of cervical cord ischemia in these patients. In some patients, however, it is likely that displacement of the cerebellar tonsils through the foramen magnum as the result of greatly elevated ICP compresses the anterior spinal artery or its penetrating branches, with resultant ischemic infarction of the upper cord (50).
Technique of Lumbar Puncture
The LP was first performed by Quincke in 1891 on children suffering from headaches in hopes to relieve their symptoms. Soon after, using CSF as a diagnostic tool became the standard way for evaluating patients with meningitis (47). The LP is generally performed with the patient in the lateral recumbent position in a fetal position with the knees flexed toward the chest, and the neck slightly flexed. Only this position allows the opening pressure to be measured. The other positions include sitting the patient upright on the edge of the bed and bending forward over a bed stand or sitting with the feet supported and chest resting on the knees.
The spinal cord typically ends as the conus medullaris at the L1 to L2 level in adults, and in children at the L3 to L4 level. The landmarks used are the anterior superior iliac crests, which correlate with the L4 to L5 interspace. The needle may be inserted between the L3 and L4, L4 and L5, or L5 and S1 interspace (51). Insertion above the L3 level may puncture the conus medullaris and should not be attempted. Also, the needle should not be inserted over a skin infection or abscess because this has the potential of inserting bacteria into the CSF. The performer of the LP should follow a sterile technique including hand washing, gloves, gown, and mask. After the anterior superior iliac spine is identified, the spinous process superior to the interspace is palpated. Prior to inserting the spinal needle, local anesthetic should be utilized, usually 2 to 3 mL of lidocaine without epinephrine deposited subcutaneously and then deeper, allowing 1 to 2 minutes for it to take effect. The needle should be inserted 1 cm below this and directed in a horizontal position toward the umbilicus to an approximate depth of 2 cm (51). During the LP, if bone is encountered, the needle should be withdrawn to the subcutaneous layer and reinserted at a slightly different angle. The needle is inserted until a “pop” is felt indicating penetration of the ligamentum flavum and presence of the needle in the subarachnoid space. The stylet is then removed and CSF obtained. A manometer to measure the CSF pressure should be attached in all cases if possible. If CSF is not obtained, rotate the needle as part of the dura may be blocking the hole of the needle. If this does not work, reinsert the stylet and advance the needle, stopping frequently to withdraw the stylet (51).
Alternative Routes of Obtaining Cerebrospinal Fluid
Cisternal, high cervical (C2), and ventricular approaches may be used to obtain CSF if a lumbar approach is contraindicated by infection or is technically impossible (1). Cisternal puncture was initially described in 1923, but it can cause vascular injuries (52,53). Spinal puncture at the level of the second cervical vertebra under fluoroscopic guidance has been suggested as a less hazardous approach than cisternal puncture, but its actual value remains unproven. Ventricular CSF may be of great diagnostic value if there is a predominantly intraventricular infection with obstructive hydrocephalus or in the presence of a ventriculoperitoneal shunt (54).
Routine Studies of Cerebrospinal Fluid
Studies routinely obtained at the time of LP include measurement of CSF pressure, gross examination of the fluid for turbidity or changes in color, measurement of CSF protein and glucose concentrations, RBC and WBC counts, Gram and/or acid-fast stains of CSF sediment, and Gram stain and bacterial culture of the fluid. Differentiation of bacterial meningitis from viral, mycobacterial, or fungal meningitis on the basis of CSF abnormalities is presumptive unless an organism is cultured or detected by antigen tests or PCR. Amounts of CSF required by most laboratories for commonly obtained determinations are listed in Table 2.1. Because clinical laboratories differ in the amounts of CSF required for individual tests, however, the clinician must determine the amounts of CSF required by the hospital laboratory for each intended test before performing the LP.
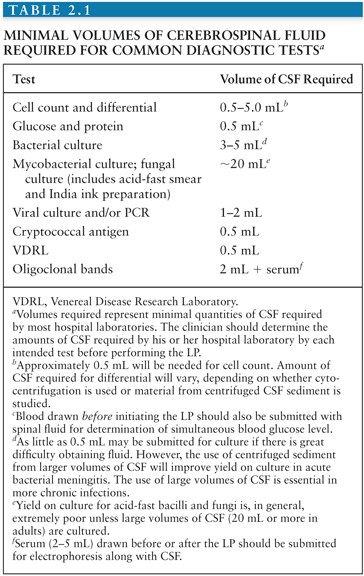
Cerebrospinal Fluid Pressure
CSF pressure must be measured in the lateral decubitus position with the head of the bed being flat. Opening CSF pressure in healthy adults lies between 50 and 195 mm CSF (1). Values higher than 200 mm are abnormal. Normal lumbar CSF pressures in neonates and premature infants are significantly lower, with mean values of 100 mm H2O and 95 mm H2O, respectively (55). CSF pressure is not affected during pregnancy (56). A CSF baseline pressure of greater than 250 mm H2O was associated with higher incidence of neurologic complaints including papilledema, hearing loss, and with mortality in AIDS patients with cryptococcal meningitis (57). Extreme elevation of CSF pressure may also herald impending brain herniation. Occasionally, CSF pressure may be normal or even low in the setting of ongoing tonsillar herniation. The falsely low readings obtained in this setting are believed to reflect occlusion of the CSF space at the foramen magnum by the herniated tonsils wedged against the lower brainstem. The possibility of complete spinal block should be kept in mind if CSF pressure falls to zero during the procedure.
Gross Appearance of the Spinal Fluid
Once CSF is obtained, it is centrifuged down to give a supernatant. Normal CSF is colorless and clear. Under pathologic conditions, CSF may become turbid, discolored, or both. The CSF may become turbid as a result of entry of cells, bacteria, or fat; it can be made turbid by as few as 200 WBCs/mm3 or 400 RBCs/mm3 (1,58). CSF containing RBCs will be grossly bloody if 6,000 or more RBCs are present per cubic millimeter, and it will be cloudy and xanthochromic or pinkish if 400 to 6,000 cells are present (1).
The yellow discoloration of the supernatant is termed xanthochromia and is often used to distinguish between a so-called bloody tap and subarachnoid hemorrhage. Xanthochromia can be assessed visually or by spectrophotometric methodology by scanning the CSF over a range of wavelengths. The discoloration is from degradation products of hemoglobin from lysis of RBCs. This usually forms 2 to 4 hours after RBCs have entered the subarachnoid space (1), which is why some experts suggest waiting at least 6 hours after the onset of headache when a subarachnoid bleed is suspected because you may get a false-negative result (58). A “traumatic tap” should clear as the CSF is collected in serial vials but not in all cases of subarachnoid hemorrhage. Xanthochromia resulting from lysis of RBCs is initially a result of oxyhemoglobin. After 12 hours, the pigment represents predominantly bilirubin (1). Visual assessment of xanthochromia can be deceitful because it may also be seen in the presence of increased amounts of protein, in metastatic melanoma, or as a consequence of systemic hyperbilirubinemia with a bilirubin level higher than 10 to 15 mg/dL. The most appropriate and sensitive way to assess xanthochromia is by spectrophotometry of the CSF to detect the hemoglobin breakdown products, oxyhemoglobin and bilirubin.
Cell Count and Differential
Enumeration and characterization of cells within spinal fluid is of crucial value in the diagnosis of CNS infections and is valuable in following the course of illness and response to treatment (Table 2.2). Improperly handled or counted CSF, however, can be a dangerous source of error. The cell count in CSF tends to decrease over time and may be falsely low if measured after 30 to 60 minutes. This decrease in cell count occurs partly because leukocytes and RBCs settle out over time if the tube of CSF is allowed to stand. In addition, however, lysis of RBCs, polymorphonuclear (PMN) leukocytes, and to a lesser extent, lymphocytes begins in vitro within 1 to 2 hours of the LP and may occasionally occur even more rapidly. WBCs also adsorb to the glass or plastic walls of the tube and are not easily dislodged by agitation. Because of these factors, the reduction in cell count that occurs over time is only partially reversible if the tube is vigorously agitated before counting. Any CSF destined for cell counts should, thus, be handled carefully and expeditiously. Similarly, where serial tubes must be counted to exclude a traumatic tap, the samples must be handled in the same manner and counted at the same time by the same person.
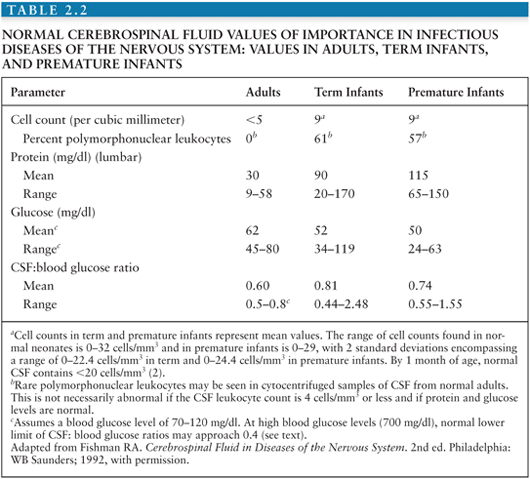
White Blood Cell Count
Quantification of numbers of cells in CSF can be carried out manually, using a Neubauer counting chamber, but this methodology is labor-intensive, time-consuming, technique-dependent, and prone to variability. Although electronic cell counters are available, they can have poor reproducibility especially if the CSF samples have low WBC counts. Novel instruments using flow cell digital imaging have excellent correlation with manual hemacytometer method and should be the method of choice (59). The accuracy of the cell count is open to question unless the specimen is examined immediately after the LP has been completed. Normally, CSF contains fewer than five cells per cubic millimeter (Table 2.2). Most of these cells are small lymphocytes (nuclear diameter about 6 to 7 µm) with scant cytoplasm. Larger numbers of PMN leukocytes are abnormal in uncentrifuged CSF. C. neoformans is similar in size to a small CSF lymphocyte, and nonbudding forms may be mistaken for these cells in the counting chamber, though not in stained cytocentrifuged or otherwise concentrated samples. Neonatal CSF usually contains 8 to 9 WBCs/mm3, and up to 32 WBCs/mm3 has been reported in the absence of disease (60) (Table 2.2).
The WBC count is usually between 1,000 and 5,000/mm3 in untreated bacterial meningitis (60), and more than 90% of patients with bacterial meningitis will have a WBC count greater than 100 cells/mm3 (61). The most common three types of viral meningitis in the United States (enterovirus, West Nile virus, and herpes simplex virus) have a median WBC count between approximately 100 and 250 cells/mm3 (25).
Differential White Blood Cell Count
A differential count of CSF leukocytes may be obtained following concentration of CSF through a Millipore filter, centrifugation of a volume (usually 5 mL) of CSF, concentration by sedimentation, or cytocentrifugation. The number of neutrophils is increased in various conditions. In adults with bacterial meningitis, neutrophils make up an average of 86.4% of cells counted, with neutrophils making up an average of 34.2% of cells counted in aseptic meningitis (1,62). In the early stages of meningitis, this distinction between bacterial and viral etiologies may not be clear because a neutrophilic pleocytosis (>50% neutrophils) may accompany early viral meningitis or encephalitis (63). Up to two thirds of enteroviral meningitis cases initially have a neutrophilic predominance (64). Within 12 to 24 hours, there is usually a shift from a neutrophilic predominance to a lymphocytic predominance, which is why some may suggest a repeat LP if the first LP was nonspecific (47). A lymphocytic pleocytosis is typically observed in patients with viral meningitis, M. tuberculosis, Borrelia burgdorferi, Treponema pallidum, or C. neoformans, as well as in neoplastic and drug-induced meningitis (63). In AIDS-associated cryptococcal meningitis, CSF pleocytosis may be absent, a finding that is associated with a worse prognosis. Only up to 30% of patients with AIDS-associated cryptococcal meningitis have a CSF WBC greater than 20 with a lymphocytic predominance (57). In tuberculous meningitis, the range of CSF pleocytosis is more commonly between 50 and 300 cells/mm3 with a lymphocytic predominance. Plasma cells and eosinophils should not be present in normal CSF (60). Increased numbers of B cells can be seen in neurosyphilis and could represent another diagnostic option (65). Eosinophilic meningitis can be caused by several parasitic infections, the most common being angiostrongyliasis, gnathostomiasis, toxocariasis, cysticercosis, schistosomiasis, baylisascariasis, and paragonimiasis (66) (Table 2.3). In addition, however, CSF eosinophilia has been reported in a wide variety of other infectious and noninfectious conditions (Table 2.3), so detection of eosinophils within the CSF is not pathognomonic of parasitic infestation (66).
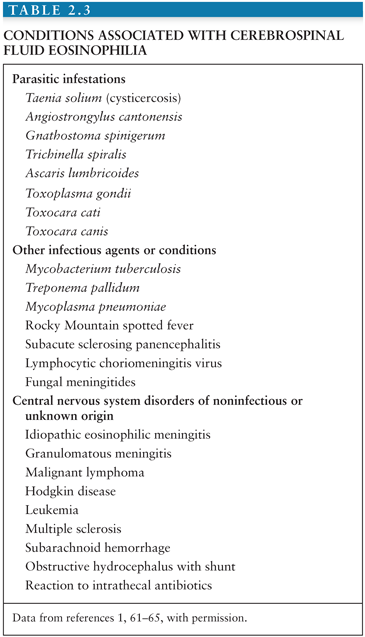
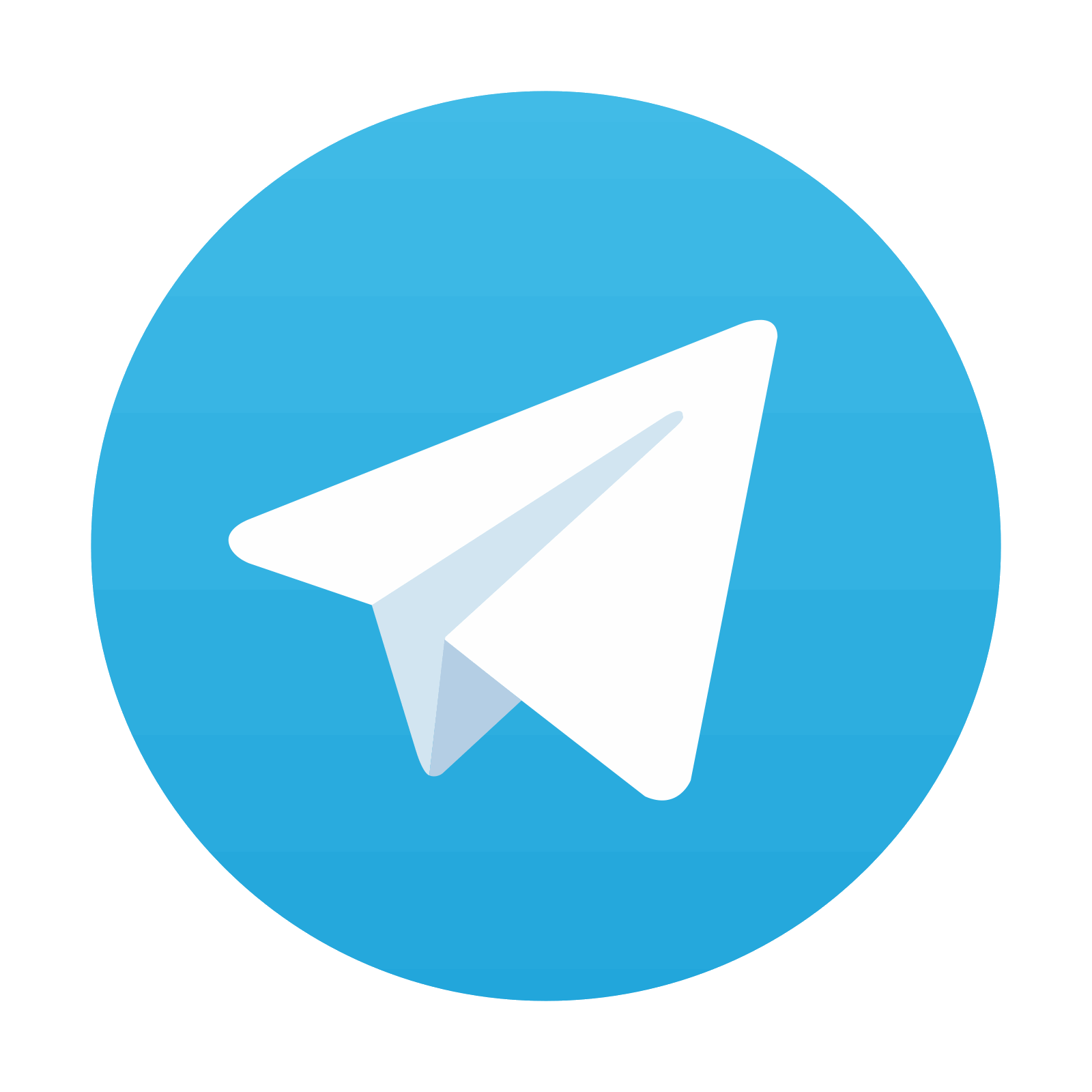
Stay updated, free articles. Join our Telegram channel
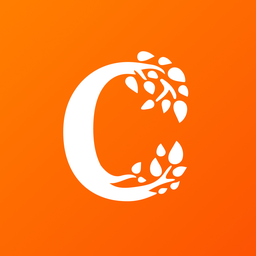
Full access? Get Clinical Tree
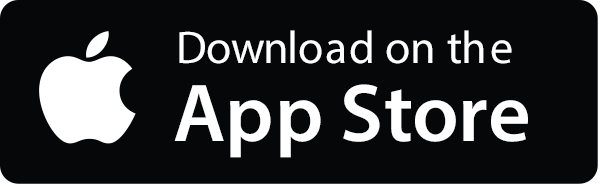
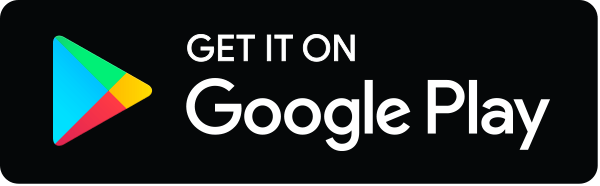