INTRODUCTION
SUMMARY
The thalassemias are the commonest monogenic diseases in man. They occur at a high gene frequency throughout the Mediterranean populations, the Middle East, the Indian subcontinent, and Myanmar, and in a line stretching from southern China through Thailand and the Malay peninsula into the island populations of the Pacific. They are also seen commonly in countries in which there has been immigration from these high-frequency populations.
There are two main classes of thalassemias, α and β, in which the α– and β-globin genes are involved, and rarer forms caused by abnormalities of other globin genes. Some extremely rare congenital and acquired thalassemia that have intact globin genes are caused by either mutations of nonglobin genes or factors yet to be elucidated. All thalassemias have in common an imbalanced rate of production of the globin chains of adult hemoglobin, excess α chains in β-thalassemia and excess β chains in α-thalassemia. Several hundred different mutations at the α– and β-globin loci have been defined as the cause of the reduced or absent output of α or β chains. The high frequency and genetic diversity of the thalassemias is related to past or present heterozygote resistance to malaria.
The pathophysiology of the thalassemias can be traced to the deleterious effects of the globin-chain subunits that are produced in excess. In β-thalassemia, excess α chains cause damage to the red cell precursors and red cells and lead to profound anemia. This causes expansion of the ineffective marrow, with severe effects on development, bone formation, and growth. The major cause of morbidity and mortality is the effect of iron deposition in the endocrine organs, liver, and heart, which results from increased intestinal absorption and the effects of blood transfusion. The pathophysiology of the α-thalassemias is different because the excess β chains that result from defective α-chain production form β4 molecules, or hemoglobin H, which is soluble and does not precipitate in the marrow. However, it is unstable and precipitates in older red cells. Hence, the anemia of α-thalassemia is hemolytic rather than dyserythropoietic.
The clinical pictures of α– and β-thalassemia vary widely, and knowledge is gradually being amassed about some of the genetic and environmental factors that modify these phenotypes.
Because the carrier states for the thalassemias can be identified and affected fetuses can be diagnosed by DNA analysis after the ninth to tenth week of gestation, these conditions are widely amenable to prenatal diagnosis. Currently, marrow transplantation is the only way in which they can be cured. Symptomatic management is based on regular blood transfusion, iron chelation therapy, and the judicious use of splenectomy. Experimental approaches to their management include the stimulation of fetal hemoglobin synthesis and attempts at somatic cell gene therapy.
Acronyms and Abbreviations:
AATAAA, the polyadenylation signal site; ATR-16, α-thalassemia chromosome 16-linked mental retardation syndrome; ATR-X, α-thalassemia X-linked mental retardation syndrome; BCL11A, B-cell lymphoma/leukemia oncogene important for γ– to β-globin switching; CAP site, a DNA site located in or near a promoter; DNase I, an enzyme used to detect DNA-protein interaction; GATA-1, a transcription factor essential for productive erythropoiesis; HPFH, hereditary persistence of fetal hemoglobin; HS, hypersensitive site to DNase I treatment; IVS, intervening sequence of a gene (i.e., an intron); KLF1, erythroid Kruppel-like factor; LCR, locus control region; MCS, multispecies conserved sequences; NFE-2, “nuclear factor, erythroid 2” is a transcription factor essential for productive erythropoiesis; PHD region, known as plant homeodomain is a DNA region with zinc finger motif commonly deleted in ATR-X α-thalassemia; RFLP, restriction fragment length polymorphism; TATA box, a DNA sequence (cis-regulatory element) found in the promoter region of genes.
DEFINITIONS AND HISTORY
In 1925, Cooley and Lee1 first described a form of severe anemia that occurred early in life and was associated with splenomegaly and bone changes. In 1932, George H. Whipple and William L. Bradford2 published a comprehensive account of the pathologic findings in this disease. Whipple coined the phrase thalassic anemia3,4 and condensed it to thalassemia, from θαλασσα (“the sea”), because early patients were all of Mediterranean background. The true genetic character of the disorder became fully appreciated after 1940. The disease described by Cooley and Lee is the homozygous state of an autosomal gene for which the heterozygous state is associated with much milder hematologic changes. The severe homozygous condition became known as thalassemia major. The heterozygous states, thalassemia trait, were designated according to their severity as thalassemia minor or minima.3,5,6,7 Later, the term thalassemia intermedia was used to describe disorders that were milder than the major form but more severe than the traits.
Thalassemia is not a single disease but a group of disorders, each resulting from an inherited abnormality of globin production.7 The conditions form part of the spectrum of diseases known collectively as the hemoglobinopathies, which can be classified broadly into two types. The first subdivision consists of conditions, such as sickle cell anemia, that result from an inherited structural alteration in one of the globin chains. Although such abnormal hemoglobins may be synthesized less efficiently or broken down more rapidly than normal adult hemoglobin, the associated clinical abnormalities result from the physical properties of the abnormal hemoglobin (Chap. 49). The second major subdivision of the hemoglobinopathies, the thalassemias, consists of inherited defects in the rate of synthesis of one or more of the globin chains. The result is imbalanced globin chain production, ineffective erythropoiesis, hemolysis, and a variable degree of anemia.
Thalassemia can be defined as a condition in which a reduced rate of synthesis of one or more of the globin chains leads to imbalanced globin-chain synthesis, defective hemoglobin production, and damage to the red cells or their precursors from the effects of the globin subunits that are produced in relative excess.7,8 Table 48–1 summarizes the main varieties of thalassemia that have been defined with certainty.
α-Thalassemia |
α0 |
α+ |
Deletion (–α) |
Nondeletion (αT) |
β-Thalassemia |
β0 |
β + |
Normal hemoglobin A2 |
Dominant |
Unlinked to β-globin genes |
δβ-Thalassemia |
(δβ)+ |
(δβ)0 |
(Aγ δβ)0 |
γ-Thalassemia |
δ-Thalassemia |
δ0 |
δ+ |
εγδβ-Thalassemia |
Hereditary Persistence of Fetal Hemoglobin |
Deletion |
(δβ)0, (Aγ δβ)0 |
Nondeletion |
Linked to β-globin genes |
Gγ β+, Aγ β+ |
Unlinked to β-globin genes |
The β-thalassemias are divided into two main varieties. In one form, β0-thalassemia, there is no β-chain production. In the other form, β+-thalassemia, there is a partial deficiency of β-chain production. The hallmark of the common forms of β-thalassemia is an elevated level of hemoglobin A2 in heterozygotes. In a less-common class of β-thalassemias, heterozygotes have normal hemoglobin A2 levels. Other rare forms include varieties of β-thalassemia intermedia that are inherited in a dominant fashion, that is, heterozygotes are severely affected, and there is a variety in which the genetic determinants are not linked to the β-globin gene cluster.7,9,10
The δβ-thalassemias are heterogeneous. In some cases, no δ or β chains are synthesized. Originally, these disorders were classified according to the structure of the hemoglobin F produced, that is, GγAγ(δβ)0– and Gγ(δβ)0-thalassemia. This classification is illogical. The conditions are best described by the globin chains that are defectively synthesized, that is, simply (δβ)+-, (δβ)0-, and (Aγδβ)0-thalassemia.7,10 In the (δβ)+-thalassemias, an abnormal hemoglobin is produced that has normal α chains combined with non-α chains consisting of the N-terminal residues of the δ chain fused to the C-terminal residues of the β chain. These fusion variants, called the Lepore hemoglobins, show structural heterogeneity.
The δ-thalassemias7,10 are characterized by reduced output of δ chains and hence reduced hemoglobin A2 levels in heterozygotes and an absence of hemoglobin A2 in homozygotes. They are of no clinical significance except that, when inherited with β-thalassemia trait, the level of hemoglobin A2 is reduced to the normal range.
A disorder characterized by defective ε-, γ-, δ-, and β-chain synthesis has been defined at the clinical and molecular level.7,10 The homozygous state for this condition, εγδβ-thalassemia, presumably is not compatible with fetal survival. It has been observed only in heterozygotes.
Hereditary persistence of fetal hemoglobin (HPFH) is a heterogeneous condition characterized by persistent fetal hemoglobin.7,9,10 It is classified into deletion and nondeletion forms. The deletion forms of HPFH can be classified, like δβ-thalassemia, as (δβ)0 HPFH and then subdivided according to the particular population in which this occurs and its associated molecular defect. In effect, the deletion forms of HPFH are very similar to β-thalassemia except for more efficient γ-chain synthesis and, therefore, less chain imbalance and a milder phenotype. The homozygous state is associated with mild thalassemic changes. In fact, the β-thalassemias and deletion forms of HPFH form a clinical continuum. The nondeletion forms of HPFH also are heterogeneous. In some cases, they are associated with mutations that involve the β-globin gene cluster and in which there is β-chain synthesis cis to the HPFH determinant. These conditions are subdivided into Gγβ+ HPFH and Aγβ+ HPFH. Again, they often are subclassified according to the population in which they occur, for example, Greek HPFH, British HPFH, and so on. Finally, a heterogeneous group of HPFH determinants is associated with very low levels of persistent fetal hemoglobin, the genetic loci of which, at least in some cases, are not linked to the β-globin gene cluster.
Because α chains are present in both fetal and adult hemoglobins, a deficiency of α-chain production affects hemoglobin synthesis in fetal and in adult life. A reduced rate of α-chain synthesis in fetal life results in an excess of γ chains, which form γ4 tetramers, or hemoglobin Bart’s. In adult life, a deficiency of α chains results in an excess of β chains, which form β4 tetramers, or hemoglobin H. Because there are two α-globin genes per haploid genome, the genetics of α-thalassemia is more complicated than that of β-thalassemia. There are two main groups of α-thalassemia determinants.7,10 First, in the α0-thalassemias (formerly called α-thalassemia 1), no α chains are produced from an affected chromosome; that is, both linked α-globin genes are inactivated. Second, in the α+-thalassemias (formerly called α-thalassemia 2), the output of one of the linked pair of α-globin genes is defective. The α+-thalassemias are subdivided into deletion and nondeletion types. Both the α0-thalassemias and deletion and nondeletion forms of α+-thalassemia are extremely heterogeneous at the molecular level. There are two major clinical phenotypes of α-thalassemia: the hemoglobin Bart’s hydrops syndrome, which usually reflects the homozygous state for α0-thalassemia, and hemoglobin H disease, which usually results from the compound heterozygous state for α0– and α+-thalassemia.
Because the structural hemoglobin variants and the thalassemias occur at a high frequency in some populations, the two types of genetic defect can be found in the same individual. The different genetic varieties of thalassemia and their combinations with the genes for abnormal hemoglobins produce a series of disorders known collectively as the thalassemia syndromes.7
EPIDEMIOLOGY AND POPULATION GENETICS
The β-thalassemias are distributed widely in Mediterranean populations, the Middle East, parts of India and Pakistan, and throughout Southeast Asia (Fig. 48–1).7,11,12 The disease is common in Tajikistan, Turkmenistan, Kyrgyzstan, and the People’s Republic of China. Because of the extensive migration from areas of high gene frequency such as the Mediterranean region (e.g., Italy, Greece), Africa, and Asia to the Americas, the α– and β-thalassemia genes and clinical disease are relatively common, especially in North, but also South, America. The β-thalassemias are rare in Africa, except for isolated pockets in West Africa, notably Liberia, and in parts of North Africa. However, β-thalassemia occurs sporadically in all racial groups and has been observed in the homozygous state in persons of pure Anglo-Saxon heritage. Thus, a patient’s racial background does not preclude the diagnosis.
The δβ-thalassemias have been observed sporadically in many racial groups, although no high-frequency populations have been defined. Similarly, the hemoglobin Lepore syndromes have been found in many populations, but, with the possible exceptions of central Italy, Western Europe, and parts of Spain and Portugal, these disorders have not been found to occur at a high frequency in any particular region.
The α-thalassemias occur widely throughout Africa, the Mediterranean countries, the Middle East, and Southeast Asia (Fig. 48–2).7,11,12 The α0-thalassemias are found most commonly in Mediterranean and Oriental populations, but are extremely rare in African and Middle Eastern populations. However, the deletion forms of α+-thalassemia occur at a high frequency throughout West Africa, the Mediterranean, the Middle East, and Southeast Asia. In United States, approximately 30 percent of Americans of African descent carry the gene α+-thalassemia. Up to 80 percent of the population of some parts of Papua New Guinea are carriers for the deletion form of α+-thalassemia. How common the nondeletion forms of α+-thalassemia are in any particular populations is uncertain, but they have been reported quite frequently in some of the Mediterranean island populations and in the Middle Eastern and Southeast Asian populations. Because the hemoglobin Bart’s hydrops syndrome and hemoglobin H disease require the action of an α0-thalassemia determinant, these disorders are found at a high frequency only in Southeast Asia and in parts of the Mediterranean region. The α-chain termination mutants, such as hemoglobin Constant Spring, seem to be particularly common in Southeast Asia. Approximately 4 percent of the population in Thailand are carriers.
In 1949, J.B.S. Haldane13 suggested that thalassemia had reached its high frequency in tropical regions because heterozygotes are protected against malaria.13 Although many population studies have tested this hypothesis, elucidation of some of the extremely complex population genetics underlying polymorphic systems such as the thalassemias has been possible only with the advent of recombinant DNA technology.
In each of the high-frequency areas for the β-thalassemias, a few common mutations and varying numbers of rare mutations are seen (see Fig. 48–1). Furthermore, in each of these regions the pattern of mutations is different, usually found in the context of different haplotypes in the associated β-globin gene cluster.11,14,15 Similar observations have been made in the α-thalassemias (see Fig. 48–2).7,11 These studies suggest the thalassemias arose independently in different populations and then achieved their high frequency by selection. Although some movement of the thalassemia genes may have resulted from drift, independent mutation and selection undoubtedly provide the overall basis for their world distribution. Early studies in Sardinia, showing that β-thalassemia is less common in the mountainous regions where malarial transmission is low, supported Haldane’s suggestion that β-thalassemia reached its high frequency because of protection against malarial infections.16 For many years these data remained the only convincing evidence for a protective effect. However, later studies using malaria endemicity data and globin-gene mapping showed a clear altitude-related effect on the frequency of α-thalassemia in Papua New Guinea. In addition, a sharp cline (a gradual change of species phenotype over a geographical area) in the frequency of α-thalassemia has been found in the region stretching south from Papua New Guinea through the island populations of Melanesia to New Caledonia. This is mirrored by a similar gradient in the distribution of malaria.17 The effect of drift and founder effect in these island populations has been largely excluded by showing that other DNA polymorphisms have a random distribution through the region, with no evidence of a cline similar to that characterizing the distribution of α-thalassemia and malaria.
Firm evidence for protection of individuals with mild forms of α+-thalassemia against Plasmodium falciparum malaria has been provided. In a case-control study performed in Papua New Guinea, the homozygous state for α+-thalassemia offered approximately 60 percent protection against hospital admittance because of serious complications of malaria, notably coma or profound anemia.18 Similar levels of protection by α-thalassemia against P. falciparum malaria have been found in several different African populations.19 However, it is becoming clear that there are complex genetic epistatic interactions between protective polymorphisms of this kind. For example, although α-thalassemia and the sickle cell trait both offer strong protection against P. falciparum malaria, in those who inherit both traits, the protection is canceled out and they are fully susceptible to the disease.20 Interactions of this type will have an important effect on the gene frequency of protective polymorphisms in countries in which more than one exists in the same population.
There is growing evidence that both immune and cellular mechanisms may underlie these protective effects of different red cell polymorphisms against malarial infection. Followup studies of cohorts of babies with α-thalassemia suggest that, in the first year of life, they are more prone to Plasmodium vivax and P. falciparum malaria. Because there is evidence for cross-immunization between these two species, it is possible that this effect induces early immunization that may result in babies with α-thalassemia being more resistant to P. falciparum malaria later in life.21 At the cellular level there is no evidence that α-thalassemia has any effect on the rates of parasite invasion and growth in red cells. However, parasitized α-thalassemic red cells are more susceptible to phagocytosis in vitro, and are less able than normal cells to form rosettes, an in vitro phenomena whereby uninfected cells bind to infected cells that is strongly associated with severity of infection, and express low levels of complement receptor 1, which is required for rosette formation.22 These highly complex immune and cellular interactions are discussed in detail in reviews.19,23,24 Although there are less data of this kind available for the β-thalassemias, there is strong indirect evidence that their high frequency has also been maintained by protection against P. falciparum malaria.
ETIOLOGY AND PATHOGENESIS
The structure and ontogeny of the hemoglobins are reviewed in Chaps. 7 and 49, respectively. Only those aspects with particular relevance to the thalassemia problem are discussed here.
Human adult hemoglobin is a heterogeneous mixture of proteins consisting of the major component hemoglobin A and the minor component hemoglobin A2, which constitutes approximately 2.5 percent of the total. In intrauterine life, the main hemoglobin is hemoglobin F. The structure of these hemoglobins is similar. Each consists of two separate pairs of identical globin chains. Except for some of the embryonic hemoglobins (see below), all normal human hemoglobins have one pair of α chains. In hemoglobin A, the α chains are combined with β chains (α2β2), in hemoglobin A2 with δ chains (α2δ2), and in hemoglobin F with γ chains (α2γ2).
Human hemoglobin shows further heterogeneity, particularly in fetal life, and this has important implications for understanding the thalassemias and for approaches to their prenatal diagnosis. Hemoglobin F is a mixture of molecular species with the formulas α2γ2136Gly and α2γ2136Ala. The γ chains containing glycine at position 136 are designated Gγ chains. The γ chains containing alanine are called Aγ chains. At birth, the ratio of molecules containing Gγ chains to those containing Aγ chains is approximately 3:1. The ratio varies widely in the trace amounts of hemoglobin F present in normal adults.
Before week 8 of intrauterine life, three embryonic hemoglobins—Gower 1 (ξ2ε2), Gower 2 (α2ε2), and Portland (ξ2 γ2)—are present. The ξ and ε chains are the embryonic counterparts of the adult α and β and γ and δ chains, respectively. ξ-Chain synthesis persists beyond the embryonic stage of development in some of the α-thalassemias. Persistent ε-chain production has not been found in any of the thalassemia syndromes. During fetal development, an orderly switch from ξ– to α-chain and from ε– to γ-chain production occurs, followed by β– and δ-chain production after birth.
Figure 48–3 shows the different human hemoglobins and the arrangements of the α-gene cluster on chromosome 16 and the β-gene cluster on chromosome 11.
Figure 48–3.
Genetic control of human hemoglobin (Hgb). The main globin gene clusters are located on chromosomes 11 and 16. At each stage of development, different genes in these clusters are activated or repressed. The different globin chains directed by individual genes are synthesized independently and combine in random fashion as indicated by the arrows.
Although some individual variability exists, the α-gene cluster usually contains one functional ξ gene and two α genes, designated α2 and α1. It also contains four pseudogenes: ψξ1, ψα1, ψα2, and θ1.9,10 These four pseudogenes are remarkably conserved among different species. Although it appears to be expressed early in fetal life, its function is unknown. It likely does not produce a viable globin chain. Each α gene is located in a region of homology approximately 4 kb long, interrupted by two small nonhomologous regions.25,26,27 The homologous regions are believed to result from gene duplication, and the nonhomologous segments are believed to arise subsequently by insertion of DNA into the noncoding regions around one of the two genes. The exons of the two α-globin genes have identical sequences. The first intron in each gene is identical. The second intron of α1 is nine bases longer and differs by three bases from that in the α2 gene.27,28,29 Despite their high degree of homology, the sequences of the two α-globin genes diverge in their 3′ untranslated regions 13 bases beyond the TAA stop codon. These differences provide an opportunity to assess the relative output of the genes, an important part of the analysis of the α-thalassemias.30,31 Production of α2 messenger RNA appears to exceed that of α1 by a factor of 1.5 to 3. ψξ1 and ξ2 genes also are highly homologous. The introns are much larger than those of α-globin genes. In contrast to the latter, IVS-1 is larger than IVS-2. In each ξ gene, IVS-1 contains several copies of a simple repeated 14-bp sequence that is similar to sequences located between the two ξ genes and near the human insulin gene. The coding sequence of the first exon of ψξ1 contains three base changes, one of which gives rise to a premature stop codon, thus making ψξ1 an inactive pseudogene.
The regions separating and surrounding the α-like structural genes have been analyzed in detail. Of particular relevance to thalassemia is the polymorphic nature of this gene cluster.32 The cluster contains five hypervariable regions: one downstream from the α1 gene, one between the ξ and ψξ genes, one in the first intron of both the ξ and ψξ genes, and one 5′ to the cluster. These regions consist of varying numbers of tandem repeats of nucleotide sequences. Taken together with single-base restriction fragment length polymorphisms (RFLPs), the variability of the α-globin gene cluster reaches a heterozygosity level of approximately 0.95. Thus, each parental α-globin gene cluster can be identified in the majority of persons. This heterogeneity has important implications for tracing the history of the thalassemia mutations.
Figure 48–3 shows the arrangement of the β-globin gene cluster on the short arm of chromosome 11. Each of the individual genes and their flanking regions have been sequenced.33,34,35,36 Like the α1 and α2 gene pairs, the Gγ and Aγ genes share a similar sequence. In fact, the Gγ and Aγ genes on one chromosome are identical in the region 5′ to the center of the large intron yet show some divergence 3′ to that position. At the boundary between the conserved and divergent regions, a block of simple sequence may be a “hot spot” for initiation of recombination events that lead to unidirectional gene conversion.
Like the α-globin genes, the β-gene cluster contains a series of single-point RFLPs, although in this case no hypervariable regions have been identified.37,38 The arrangement of RFLPs, or haplotypes, in the β-globin gene cluster falls into two domains. The 5′ side of the β gene, spanning approximately 32 kb from the ε gene to the 3′ end of the ψβ gene, contains three common patterns of RFLPs. The region encompassing about 18 kb to the 3′ side of the β-globin gene also contains three common patterns in different populations. Between these regions is a sequence of about 11 kb in which there is randomization of the 5′ and 3′ domains; hence, a relatively higher frequency of recombination can occur.38 The β-globin gene haplotypes are similar in most populations but differ markedly in individuals of African origin. These findings suggest the haplotype arrangements were laid down very early during evolution. The findings are consistent with data obtained from mitochondrial DNA polymorphisms pointing to the early emergence of a relatively small population from Africa with subsequent divergence into other racial groups.39 Again, they are extremely useful for analyzing the population genetics and history of the thalassemia mutations.
The regions flanking the coding regions of the globin genes contain a number of conserved sequences essential for their expression.28,33 The first conserved sequence is the TATA box, which serves accurately to locate the site of transcription initiation at the CAP site, usually about 30 bases downstream. It also appears to influence the rate of transcription. In addition, two so-called upstream promoter elements are present. A second conserved sequence, the CCAAT box, is located 70 or 80 bp upstream. The third conserved sequence, the CACCC homology box, is located further 5′, approximately 80 to 100 bp from the CAP site. It can be either inverted or duplicated. These promoter sequences also are required for optimal transcription. Mutations in this region of the β-globin gene cause its defective expression and these findings provide the foundation for understating regulation of other human genes. The globin genes also have conserved sequences in their 3′ flanking regions, notably AATAAA, which is the polyadenylation signal site.
Figure 48–4 summarizes the mechanism of globin gene expression. The primary transcript is a mRNA precursor containing both intron and exon sequences. During its stay in the nucleus, it undergoes a good deal of processing that entails capping the 5′ end and polyadenylation of the 3′ end, both of which probably serve to stabilize the transcript (Chap. 10). The intervening sequences are removed from the mRNA precursor in a complex two-stage process that relies on certain critical sequences at the intron–exon junctions.
The method by which globin gene clusters are regulated is important to understanding the pathogenesis of the thalassemias. Many details remain to be determined, but studies performed over the last few years have provided at least an outline of some of the major mechanisms of globin gene regulation.7,9,40,41,42
Most of the DNA within cells that is not involved in gene transcription is packaged into a compact form that is inaccessible to transcription factors and RNA polymerase. Transcriptional activity is characterized by a major change in the structure of the chromatin surrounding a particular gene. These alterations in chromatin structure can be identified by enhanced sensitivity to exogenous nucleases. Erythroid lineage-specific nuclease-hypersensitive sites are found at several locations in the β-globin gene cluster, which vary during different stages of development. In fetal life, these sites are associated with the promoter regions of all four globin genes. In adult erythroid cells, the sites associated with the γ genes are absent. The methylation state of the genes plays an important role in their ability to be expressed. In human and other animal tissues, the globin genes are extensively methylated in nonerythroid organs and are relatively undermethylated in hematopoietic tissues. Changes in chromatin configuration around the globin genes at different stages of development are reflected by alterations in their methylation state.
In addition to the promoter elements, several other important regulatory sequences have been identified in the globin gene clusters. For example, several enhancer sequences thought to be involved with tissue-specific expression have been identified. Their sequences are similar to the upstream activating sequences of the promoter elements. Both consist of a number of “modules,” or motifs, that contain binding sites for transcriptional activators or repressors. The enhancer sequences are thought to act by coming into spatial apposition with the promoter sequences to increase the efficiency of transcription of particular genes. It now is clear that transcriptional regulatory proteins may bind to both the promoter region of a gene and to the enhancer. Some of these transcriptional proteins, GATA-1 and NFE-2, for example, appear to be largely restricted to hematopoietic tissues.40 These proteins may bring the promoter and the enhancer into close physical proximity, permitting transcription factors bound to the enhancer to interact with the transcriptional complex that forms near the TATA box. At least some of these hematopoietic gene transcription factors likely will be developmental-stage specific.
Another set of erythroid-specific nuclease-hypersensitive sites is located upstream from the embryonic globin genes in both the α– and β-gene clusters. These sites mark the regions of particularly important control elements. In the case of the β-globin gene cluster, the region is marked by five hypersensitive sites to DNase I treatment (HS) (an enzyme used to detect DNA-protein interaction).40 The most 5′ site (HS5) does not show tissue specificity. HS1 through HS4, which together form the locus control region (LCR), are largely erythroid-specific. Each of the regions of the LCR contains a variety of binding sites for erythroid transcription factors. The precise function of the LCR is not known, but it is undoubtedly required to establish a transcriptionally active domain spanning the entire globin gene cluster. The α-globin gene cluster also has a major regulatory element of this kind, in this case HS40.41 This forms part of four highly conserved noncoding sequences, or multispecies conserved sequences (MCSs), called MSC-R1-R4; of these elements only MSC-R2, that is HS40, is essential for α-globin gene expression. Although deletions of this region inactivate the entire α-globin gene cluster, its action must be fundamentally different from that of the β-globin LCR because the chromatin structure of the α-gene cluster is in an open conformation in all tissues.
Some forms of thalassemia result from deletions involving these regulatory regions. In addition, the phenotypic effects of deletions of these gene clusters are strongly positional, which may reflect the relative distance of particular genes from the LCR and HS40.
One particularly important aspect of human globin genes is regulation of the switch from fetal to adult hemoglobin. Because many of the thalassemias and related disorders of the β-globin gene cluster are associated with persistent γ-chain synthesis, a full understanding of their pathophysiology must include an explanation for this important phenomenon, which plays a considerable role in modifying their phenotypic expression.
The complex topic of hemoglobin switching has been the subject of several extensive reviews.7,42 β-Globin synthesis commences early during fetal life, at approximately 8 to 10 weeks’ gestation. β-Globin synthesis continues at a low level, approximately 10 percent of the total non–α-globin chain production, up to approximately 36 weeks’ gestation, after which it is considerably augmented. At the same time, γ-globin chain synthesis starts to decline so that, at birth, approximately equal amounts of γ– and β-globin chains are produced. Over the first year of life, γ-chain synthesis gradually declines. By the end of the first year, γ-chain synthesis amounts to less than 1 percent of the total non–α-globin chain output. In adults the small amount of hemoglobin F is confined to an erythrocyte population called F cells.
How this series of developmental switches is regulated is not clear. The process is not organ specific but is synchronized throughout the developing hematopoietic tissues. Although environmental factors may be involved, the bulk of experimental evidence suggests some form of “time clock” is built into the hematopoietic stem cell. At the chromosomal level, regulation appears to occur in a complex manner involving both developmental stage-specific trans-activating factors and the relative proximity of the different genes of the β-globin gene cluster to LCR. Some of the elements involved in the stage-specific regulation of human globin genes have been identified. KLF1 (erythroid Kruppel-like factor), a developmental stage–enriched protein, activates human β-globin gene expression and is involved in human γ– to β-globin gene switching.43 More recently BCL11A and MYB have also been identified as being involved in this process.42
Fetal hemoglobin synthesis can be reactivated at low levels in states of hematopoietic stress and at higher levels in certain hematologic malignancies, notably juvenile myeloid leukemia. However, high levels of hemoglobin F production are seen consistently in adult life only in the hemoglobinopathies.
MOLECULAR BASIS OF THE THALASSEMIAS
Once cloning and sequencing of globin genes from patients with many different forms of thalassemia were possible, the wide spectrum of mutations underlying these conditions became clear. A picture of remarkable heterogeneity has emerged. For more extensive coverage of this topic, the reader is referred to several monographs and reviews.7,9,10,44,45,46
β-thalassemia is extremely heterogeneous at the molecular level.7 More than 200 different mutations have been found in association with the β-thalassemia phenotype.7 Broadly, they fall into deletions of the β-globin gene and nondeletional mutations that may affect the transcription, processing, or translation of β-globin messenger (Table 48–2 and Fig. 48–5). Each major population group has a different set of β-thalassemia mutations, usually consisting of two or three mutations forming the bulk and large numbers of rare mutations. Because of this distribution pattern, only approximately 20 alleles account for the majority of all β-thalassemia determinants (see Fig. 48–1).
Figure 48–5.
Classes of mutations that underlie β-thalassemia. C, CAP site; FS, frameshift; I, initiation site; NS, nonsense mutation; POLY A, polyA addition site mutation; PR, promoter; SPL, splicing mutation. For a complete list see ref. 304.
β0– or β+-Thalassemia |
Transcription |
Deletions |
Insertions |
Promoter |
5′-UTR |
Processing of mRNA |
Junctional |
Consensus splicing sequences |
Cryptic splice sites in introns |
Cryptic splice sites in exons |
Poly (A) addition site |
Translation |
Initiation |
Nonsense |
Frameshift |
Posttranslational stability |
Unstable β-chain variants |
Normal hemoglobin A2 β-thalassemia |
β-Thalassemia and δ-thalassemia, cis or trans |
“Silent” β-thalassemia |
Some promoter mutations |
CAP +1, CAP +3, etc. |
5′ UTR |
Some splice mutations |
Dominant β-thalassemia |
Mainly point mutations or rearrangements in exon 3 |
Other unstable variants |
At least 17 different deletions affecting only the β genes have been described. With one exception, the deletions are rare and appear to be isolated, single events. The 619-bp deletion at the 3′ end of the β gene is more common,48 but even that is restricted to the Sind and Gujarati populations of Pakistan and India, where it accounts for approximately 50 percent of β-thalassemia alleles.48 The Indian 619-bp deletion removes the 3′ end of the β gene but leaves the 5′ end intact. Many of the other deletions remove the 5′ end of the gene and leave the δ gene intact.49,50,51,52,53 Homozygotes for these deletions have β0-thalassemia. Heterozygotes for the Indian deletion have increased hemoglobin A2 and F levels identical to those seen in heterozygotes for the other common forms of β-thalassemia. Heterozygotes for the other deletions all have unusually high hemoglobin A2 levels.7 Increased δ-chain production results from increased δ-gene transcription in cis to the deletion, possibly as a result of reduced competition from the deleted 5′ β gene for transcription factors.
Several different base substitutions involve the conserved sequences upstream from the β-globin gene.7 In every case, the phenotype is β+-thalassemia, although considerable variability exists in the clinical severity associated with different mutations of this type. Several mutations, at positions –88 and –87 relative to the mRNA CAP site, for example,54,55 are close to the CCAAT box, whereas others lie within the TATA box homology.56,57,58,59
Some mutations upstream from the β-globin gene are associated with even more subtle alterations in phenotype. For example, a C→T substitution at position –101, which involves one of the upstream promoter elements, is associated with “silent” β-thalassemia, that is, a completely normal (“silent”) phenotype that can be identified only by its interaction with more severe forms of β-thalassemia in compound heterozygotes.60 A single example of an A→C substitution at the CAP site (+1) was described in an Asian Indian who, despite being homozygous for the mutation, appeared to have the phenotype of the β-thalassemia trait.61
Upstream regulatory mutations confirm the importance of the role of conserved sequences in this region as regulators of the transcription of the β-globin genes and provide the basis for some of the mildest forms of β-thalassemia, particularly those in African populations, and for some varieties of “silent” β-thalassemia.
One surprise about β-thalassemia has been the remarkable diversity of the single-base mutations that can interfere with the intranuclear processing of mRNA.
The boundaries of exons and introns are marked by invariant dinucleotides, GT at the 5′ (donor) and AG at the 3′ (receptor) sites. Single-base changes that involve either of these splice junctions totally abolish normal RNA splicing and result in the β0-thalassemia phenotype.7,62,63,64,65,66
Highly conserved sequences involved in mRNA processing surround the invariant dinucleotides at the splice junctions. Different varieties of β-thalassemia involve single-base substitutions within the consensus sequence of the IVS-1 donor site.55,58,63,64,65,66,67,68,69 These mutations are particularly interesting because of the remarkable variability in their associated phenotypes. For example, substitution of the G in position 5 of IVS-1 by C or T results in severe β+-thalassemia.55 On the other hand, a T→C change at position 6, found commonly in the Mediterranean region,70 results in a very mild form of β+-thalassemia. The G→C change at position 5 has also been found in Melanesia and appears to be the most common cause of β-thalassemia in Papua New Guinea.71
RNA processing is affected by mutations that create new splice sites within either introns or exons. Again, these lesions are remarkably variable in their phenotypic effect, depending on the degree to which the new site is utilized compared with the normal splice site. For example, the G→A substitution at position 110 of IVS-1, which is one of the most common forms of β-thalassemia in the Mediterranean region, leads to only approximately 10 percent splicing at the normal site and hence results in a severe β+-thalassemia phenotype.72,73 Similarly, a mutation that produces a new acceptor site at position 116 in IVS-1 results in little or no β-globin mRNA production and the β0-thalassemia phenotype.74 Several mutations that generate new donor sites within IVS-2 of the β-globin gene have been described.55,68
Another mechanism for abnormal splicing is activation of donor sites within exons (Fig. 48–6). For example, within exon 1 is a cryptic donor site in the region of codons 24 through 27. This site contains a GT dinucleotide. An adjacent substitution that alters the site so that it more closely resembles the consensus donor splice site results in its activation, even though the normal site is active. Several mutations in this region can activate this site so that it is utilized during RNA processing, with the production of abnormal mRNAs.75,76,77,78 Three of the substitutions—A→G in codon 19, G→A in codon 26, and G→T in codon 27—result in reduced production of β-globin mRNA and an amino acid substitution so that the mRNA that is spliced normally is translated into protein. The abnormal hemoglobins produced are hemoglobins Malay, E, and Knossos, respectively, all of which are associated with a β-thalassemia phenotype, presumably as a result of reduced overall output of normal mRNA (Fig. 48–6). A variety of other cryptic splice mutations within introns and exons have been described.44
Another class of processing mutations involves the polyadenylation signal site AAUAAA in the 3′ untranslated region of β-globin mRNA.79,80,81 For example, a T→C substitution in this sequence leads to only one-tenth the normal amount of β-globin mRNA and hence the severe β+-thalassemia phenotype.79
Base substitutions that change an amino acid codon into a chain termination codon, that is, nonsense mutations, prevent translation of the mRNA and result in β0-thalassemia. Many substitutions of this type have been described.7,44 For example, a codon 17 mutation is common in Southeast Asia,82,83 and a codon 39 mutation occurs at a high frequency in the Mediterranean region.84,85
The insertion or deletion of one, two, or four nucleotides in the coding region of the β-globin gene disrupts the normal reading frame and results, upon translation of the mRNA, in the addition of anomalous amino acids until a termination codon is reached in the new reading frame. Several frameshift mutations of this type have been described.7,44 Two mutations—the insertion of one nucleotide between codons 8 and 9 and a deletion of four nucleotides in codons 41 and 42—are common in Asian Indians.63 The latter deletions are found frequently in different populations in Southeast Asia.83
An unusual β+-thalassemia was described in a patient from the Czech Republic in whom a full-length L1 transposon was inserted into the second intron of β-globin, creating a β+-thalassemia phenotype by an undefined molecular mechanism.86
Families in which a picture indistinguishable from moderately severe β-thalassemia has segregated in mendelian dominant fashion have been reported sporadically.87,88 Because this condition often is characterized by the presence of inclusion bodies in the red cell precursors, it has been called inclusion body β-thalassemia. However, because all severe forms of β-thalassemia have inclusions in the red cell precursors, the term dominantly inherited β-thalassemia is preferred.7,89 Sequence analysis has shown that these conditions are heterogeneous at the molecular level, but that many involve mutations of exon 3 of the β-globin gene. The mutations include frameshifts, premature chain termination mutations, and complex rearrangements that lead to synthesis of truncated or elongated and highly unstable β-globin gene products.7,89,90,91,92,93 The most common mutation of this type is a GAA→TAA change at codon 121 that leads to synthesis of a truncated β-globin chain.94 Although an abnormal β-chain product from loci affected by mutations of this type is unusual, many of these conditions are designated as hemoglobin variants.
The reason why mutations occurring in exons 1 and 2 produce the classic form of recessive β-thalassemia whereas the bulk of the dominant thalassemias result from mutations in exon 3 has become clearer. In the former case, very little abnormal β-globin mRNA is found in the cytoplasm of the red cell precursors, whereas exon 3 mutations are associated with full-length but abnormal mRNA accumulation. The different phenotypes of these premature termination codons have been suggested to reflect a phenomenon called nonsense-mediated RNA decay, a surveillance system to prevent transport of mRNA coding for truncated peptides. Presumably this process is active in the case of exon 1 or 2 mutations, in which affected mRNAs are degraded, but is not active in the case of exon 3 mutations.95,96,97 A complete list of the mutations that underlie the dominant β-thalassemias is given in reference 44.
Some β-globin chain variants are highly unstable but are capable of forming a viable tetramer. The resulting unstable hemoglobins may precipitate in the red cell precursors or in the blood, giving rise to a spectrum of conditions ranging from dominantly inherited β-thalassemia to a hemolytic anemia similar to the anemia associated with other unstable hemoglobins. The first unstable hemoglobin to be described was hemoglobin Indianapolis.98 Its structure was characterized by DNA analysis performed on stored autopsy material; however, the original description proved to be incorrect.99
A number of extremely mild β-thalassemia alleles are either silent or almost unidentifiable in heterozygotes (see Table 48–2). Some alleles are in the region of the promoter boxes of the β-globin gene, but others involve the CAP sites or the 5′ or 3′ untranslated regions.7,44 These alleles usually are identified by finding a form of β-thalassemia intermedia in which one parent has a typical thalassemia trait and the other parent appears to be normal but, in fact, is a carrier of one of the mild β-thalassemia alleles.
Several family studies suggest the existence of mutations that result in the β-thalassemia phenotype but do not segregate with the β-globin genes100; however, their molecular basis has not been determined. Further evidence for the existence of novel mutations of this type can be found in reference 7.
In several forms of β-thalassemia, the hemoglobin A2 level is normal in heterozygotes. Some cases result from “silent” β-thalassemia alleles, whereas others reflect the coinheritance of β– and δ-thalassemia.7
The δβ-thalassemias are classified into the (δβ)+– and (δβ)0-thalassemias (Table 48–3). The (δβ)0-thalassemias are further divided into (δβ)0-thalassemia, in which both the δ– and β-globin genes are deleted, and (Aγδβ)0-thalassemia, in which the Gγ, δ, and β genes are deleted. Because many different deletion forms of δβ-thalassemia have been described, they are further classified according to the country in which they were first identified (Table 48–3).
(δβ)+-Thalassemia |
Hgb Lepore thalassemia |
Hgb Lepore Washington-Boston |
Hgb Lepore Hollandia |
Hgb Lepore Baltimore |
Phenocopies of (δβ)+-thalassemia |
Sardinian δβ-thalassemia |
Corfu δβ-thalassemia |
Chinese δβ-thalassemia |
β-Thalassemia with δ-thalassemia |
(δβ)°-Thalassemia |
Sicilian |
Indian |
Japanese |
Spanish |
Black |
Eastern European |
Macedonian |
Turkish |
Laotian |
Thai |
(Aγδβ)°-Thalassemia |
Indian |
German |
Cantonese |
Turkish |
Malay 2 |
Belgian |
Black |
Chinese |
Yunnanese |
Thai |
Italian |
Nearly all these conditions result from deletions involving varying lengths of the β-globin gene cluster. Many different varieties have been described in different populations (see Table 48–3), although their heterozygous and homozygous phenotypes are very similar.7 Rare forms of these conditions result from more complex gene rearrangements. For example, one form of (Aγδβ)0-thalassemia, found in Indian populations, does not result from a simple linear deletion but rather from a complex rearrangement with two deletions, one affecting the Aγ gene and the other the δ and β genes. The intervening region is intact but inverted.101 Figure 48–7 illustrates some of these conditions.
Figure 48–7.
Some deletions responsible for the β– and δβ-thalassemias and hereditary persistence of fetal hemoglobin. For a complete list see reference 304.
The (δβ)+-thalassemias usually are associated with the production of structural hemoglobin variants called Lepore.102 Hemoglobin Lepore contains normal α chains and non-α chains that consist of the first 50 to 80 amino acid residues of the δ chains and the last 60 to 90 residues of the normal C-terminal amino acid sequence of the β chains. Thus, the Lepore non-α chain is a β-fusion chain. Several different varieties of hemoglobin Lepore have been described—Washington-Boston, Baltimore, and Hollandia—in which the transition from δ to β sequences occurs at different points.7 The fusion chains probably arose by nonhomologous crossing over between part of the δ locus on one chromosome and part of the β locus on the complementary chromosome (Fig. 48–8). This event results from misalignment of chromosome pairing during meiosis so that a δ-chain gene pairs with a β-chain gene instead of with its homologous partner.103 Figure 48–8 shows such a mechanism should give rise to two abnormal chromosomes: the first, the Lepore chromosome, will have no normal δ or β loci but simply a δβ fusion gene. Opposite the homologous pairs of chromosomes should be an anti-Lepore (βδ) fusion gene and normal δ and β loci. A variety of anti–Lepore-like hemoglobins have been discovered, including hemoglobins Miyada, P-Congo, Lincoln Park, and P-Nilotic.7 All the hemoglobin Lepore disorders are characterized by a severe form of δβ-thalassemia. The output of the γ-globin genes on the chromosome with the δβ fusion gene is not increased sufficiently to compensate for the low output of the δβ fusion product. The reduced rate of production of the δβ fusion chains of hemoglobin Lepore presumably reflects the fact that its genetic determinant has the δ gene promoter region, which is structurally different from the β-globin gene promoter and is associated with a reduced rate of transcription of its gene product.
A heterogeneous group of nondeletion δβ-thalassemias has been described, most resulting from two mutations in the εγδβ-globin gene cluster (see Table 48–3). Strictly speaking, they are not all δβ-thalassemias, but they often appear in the literature under this title because their phenotypes resemble the deletion forms of (δβ)0-thalassemia. In the Sardinian form of δβ-thalassemia, the β-globin gene has the common Mediterranean codon 39 nonsense mutation that leads to an absence of β-globin synthesis. The relatively high expression of the Aγ gene in cis gives this condition the δβ-thalassemia phenotype because of a point mutation at position –196 upstream from the Aγ gene (see “Hereditary Persistence of Fetal Hemoglobin” below). The phenotypic picture, in which heterozygotes have 15 to 20 percent hemoglobin F and normal hemoglobin A2 levels, is identical to that of δβ-thalassemia.103 Another condition having the β-thalassemia phenotype, with greater than 20 percent hemoglobin F in heterozygotes, has been described in a Chinese patient in whom defective β-globin chain synthesis appears to result from an A→G change in the ATA sequence in the promoter region of the β-globin gene.104 The increased γ-chain synthesis, which appears to involve both Gγ and Aγ cis to this mutation, remains unexplained. A disorder originally called δβ-thalassemia has been described in the Corfu population.105,106 The condition results from two mutations in the β-globin gene cluster: first, a 7201-bp deletion that starts in the δ-globin gene, IVS-2, position 818 to 822, and extends upstream to a 5′ breakpoint located 1719 to 1722 bp 3′ to the ψβ-gene termination codon; and second, a G→A mutation at position 5 in the donor site consensus region of IVS-1 of the β-globin gene. The output from this chromosome consists of relatively high levels of γ chains with very low levels of β chains. The condition resembles δβ-thalassemia in the homozygous state, with almost 100 percent hemoglobin F, traces of hemoglobin A, but no hemoglobin A2. Heterozygotes have only slightly elevated hemoglobin F levels, with a phenotype similar to “normal A2β-thalassemia.”
These rare conditions107,108,109,110,111,112,113 result from long deletions that begin upstream from the β-gene complex 55 kb or more 5′ to the ε gene and terminate within the cluster (see Fig. 48–7). In two cases, designated Dutch110,111 and English,112 the deletions leave the β-globin gene intact, but no β-chain production occurs even though the gene is expressed in heterologous systems.
The molecular basis for inactivation of the β-globin gene cis to these deletions was clarified by the discovery of the LCR approximately 50 kb upstream from the εγδβ-globin gene cluster (see “Genetic Control and Synthesis of Hemoglobin” above). Removal of this critical regulatory region seems to completely inactivate the downstream globin gene complex. The Hispanic form of εγδβ-thalassemia113 results from a deletion that includes most of the LCR, including four of the five DNase-1-hypersensitive sites. These lesions appear to close down the chromatin domain that usually is open in erythroid tissues and delay replication of the β-globin genes in the cell cycle. Thus, although they are rare, the lesions have been of considerable importance because analysis of the Dutch deletion first pointed to the possibility of a major control region upstream from the β-like-globin gene cluster and ultimately led to the discovery of the β-globin LCR.
This heterogeneous group of conditions produces phenotypes very similar to those of the δβ-thalassemias, except that defective β-chain production appears to be almost, but in some forms not completely, compensated by persistent γ-chain production. These conditions are best classified into deletion and nondeletion forms (Table 48–4). In the past, the conditions were classified into pancellular and heterocellular varieties, depending on the intercellular distribution of fetal hemoglobin. However, this subdivision now appears to bear little relevance to their molecular basis and probably relates more to the particular level of fetal hemoglobin and how its cellular distribution is determined.7
Deletion (Pancellular*) |
(δβ)0 |
Black (HPFH 1) |
Ghanaian (HPFH 2) |
Indian (HPFH 3) |
Italian (HPFH 4 and 5) |
Vietnamese (HPFH 6) |
Gγ (Aγ β)+ (Hgb Kenya) |
Nondeletion |
Linked to β-globin gene cluster (pancellular*) |
Gγ β+ |
Black Gγ-202 C→G |
Tunisian Gγ-200+C |
Black/Sardinian Gγ-175 T→C |
Japanese Gγ-114 C→T |
Australian Gγ-114 C→G |
Aγ β+ |
Greek/Sardinian/Black Aγ-117 G→A |
British Aγ-198 T→C |
Black Aγ-202 C→T |
Italian/Chinese Aγ-196 C→T |
Brazilian Aγ-195 C→G |
Black Aγ-175 T→C |
Black Aγ-114 to –102 (del) |
Georgia Aγ-114 C→T |
Gγ Aγ β+ |
Linked to β-globin gene cluster (heterocellular*) |
Atlanta |
Czech |
Seattle |
Others (including some cases of Gγ-158 T→C) |
Unlinked to β-globin gene cluster (heterocellular*) |
Chromosome 6 |
Others |
The deletion forms of HPFH are heterogeneous (see Fig. 48–7). The two African varieties result from extensive deletions of similar length (<70 kb) but with staggered ends, differing phenotypically only in the proportions of Gγ and Aγ chains produced.114 Another type of HPFH results from misalignment during crossing over between the Aγ– and β-globin genes, resulting in production of Aγβ fusion genes (see Fig. 48–8) that combine with α chains to form the hemoglobin variant called hemoglobin Kenya.115,116 Hemoglobin Kenya is associated with an increased output of hemoglobin F, although at a lower level than in the deletion forms of HPFH. A theory that adequately explains the phenotypic differences between δβ-thalassemia and the deletion forms of HPFH has not been developed.7
The nondeletion determinants of HPFH can be classified into those that map within the β-globin gene cluster and those that segregate independently. The former are subdivided into Gγβ+ and Aγβ+ varieties, indicating persistent Gγ– or Aγ-chain synthesis in association with β-globin production directed by the β gene cis (on the same chromosome) to the HPFH determinant. Analysis of the overexpressed γ genes revealed in each case a single-base substitution in the region immediately upstream from the transcription start site.7,117,118,119,120 Clustering of these substitutions and lack of similar changes in normal γ genes suggest they are responsible for persistent hemoglobin F production (Fig. 48–9). This region of DNA likely is involved in binding of trans-acting proteins involved in the normal developmental repression of γ-gene expression, either by decreasing the affinity for an inhibitory factor normally present in adult life or by increasing the affinity for a factor promoting gene expression. The most common of these conditions are Greek Aγβ+ HPFH and a form of Gγβ+ HPFH, which has been found in several different African populations. If the upstream point mutations associated with persistent γ-chain production occur on the same chromosome as β-globin genes that carry β0-thalassemia mutations, the clinical phenotype is converted from HPFH to δβ-thalassemia, albeit with different hemoglobin A2 levels.
In some cases, other nondeletional forms of HPFH have been related to small structural changes in the β-globin gene cluster (see Table 48–4). Although strictly speaking not a true form of HPFH, because even in homozygotes it may not be associated with increased hemoglobin F levels, the T→C polymorphism at position –158 to the Gγ-globin gene121 might be associated with an increased output of hemoglobin F under conditions of erythropoietic stress.
Other forms of HPFH are characterized by the persistence of low levels of fetal hemoglobin production distributed in a heterocellular manner. In all populations studied, a small proportion of individuals have an increased amount of hemoglobin F and F cells, that is, red cells that can be detected when blood films are treated with antibodies against hemoglobin F. Although this condition originally was called the Swiss form of HPFH because it was first recognized in Swiss army recruits,122 it is observed in every racial group. Using a variety of genetic approaches, it has become clear that a number of genes may be involved in the generation of heterocellular HPFH, including loci at Xp22.2-p22.3,6q23,8q, and 2p15123,124,125,126,127,128; the latter linkage has been identified as the oncogene BCL11A. The mechanism whereby these different loci affect the level of F cells in normal individuals and increase their levels in conditions like thalassemia and sickle cell anemia remain to be determined, but their coinheritance with these conditions may have an extremely beneficial effect of their associated phenotypes.129
Several point mutations and deletions that reduce δ-globin synthesis have been described. They are summarized in reference 7.
Table 48–5 summarizes the different classes of α-thalassemia mutations. The α-globin gene haplotype can be written αα, indicating the α1 and α2 genes, respectively. A normal individual has the genotype αα/αα. A deletion involving one (–α) or both (– –) α genes can be further classified based on its size, written as a superscript; thus, –α3.7 indicates a deletion of 3.7 kb including one α gene. When the sizes of the deletions are not established, a superscript describing their geographic or family origin is useful; thus, – – MED describes a deletion of both α genes first identified in individuals of Mediterranean origin. In thalassemia haplotypes in which both genes are intact, that is, nondeletion lesions, the nomenclature αNDα is given, with the superscript ND indicating the gene is thalassemic. However, when the precise molecular defect is known, as in hemoglobin Constant Spring, for example, αNDα can be replaced by the more informative αCSα. The molecular pathology and population genetics of the α-thalassemias have been the subject of several extensive reviews.7,41,45,130,131
α0-Thalassemia |
Deletions involving both α-globin genes |
Deletions downstream from α2 gene |
Truncations of telomeric region of 16p |
Deletions of HS40 region |
α+-Thalassemia |
Deletions involving α2 or α1 genes |
Point mutations involving α2 or α1 genes |
mRNA processing |
Splice site |
Poly(A) signal |
mRNA translation |
Initiation |
Nonsense, frameshift |
Termination |
Posttranslational |
Unstable α-globin variants |
α-Thalassemia Mental Retardation |
ATR-16 |
Deletions or telomeric truncations of 16p |
Translocations |
ATR-X |
Mutations of ATR-X |
Deletions |
Splice site |
Missense |
Nonsense |
Many deletions that involve both α genes, and therefore abolish α-chain production from the affected chromosome, have been described (Fig. 48–10).7 Several of the 3′ breakpoints fall within a 6- to 8-kb region at the 3′ end of the α
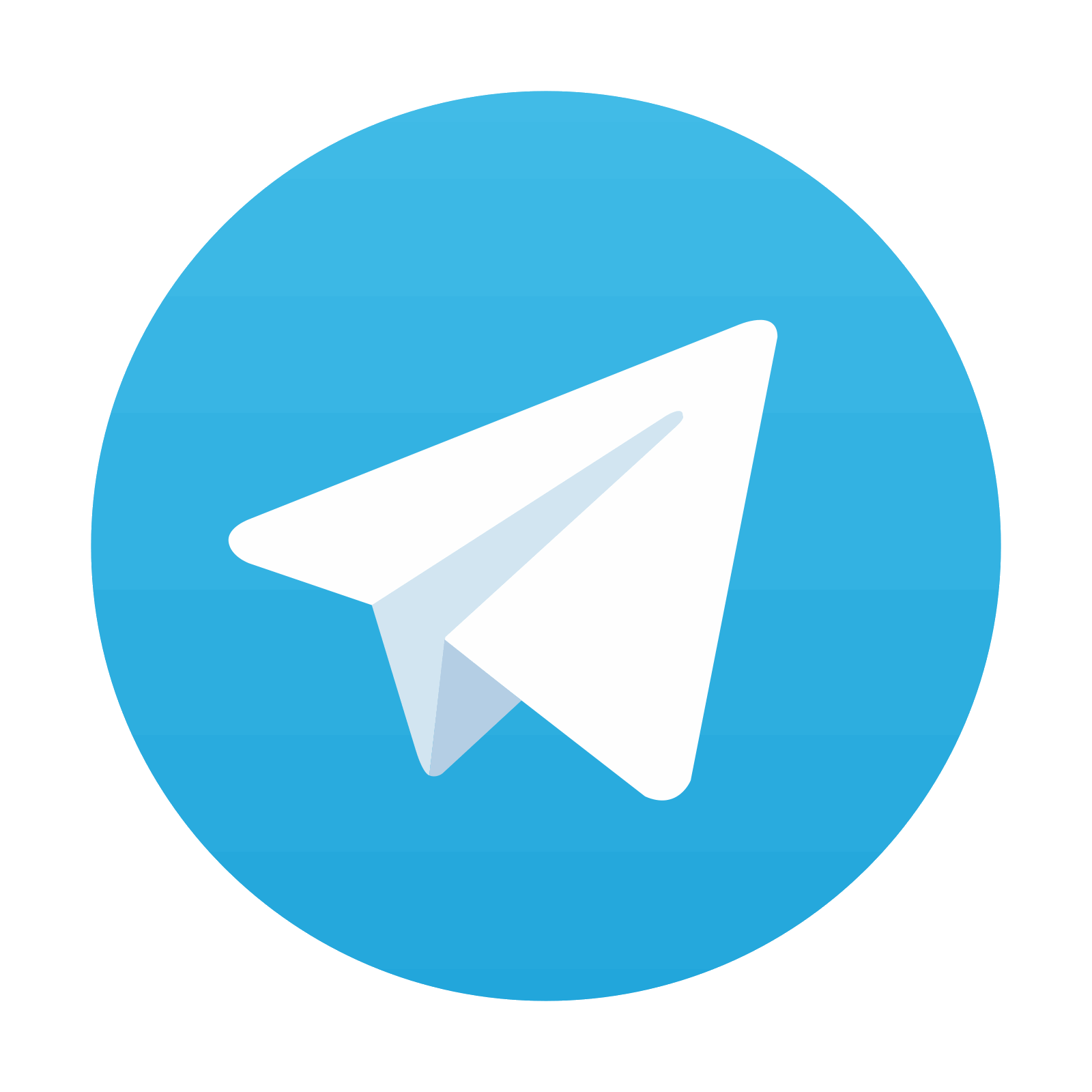
Stay updated, free articles. Join our Telegram channel
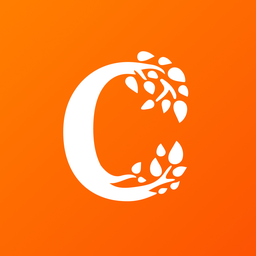
Full access? Get Clinical Tree
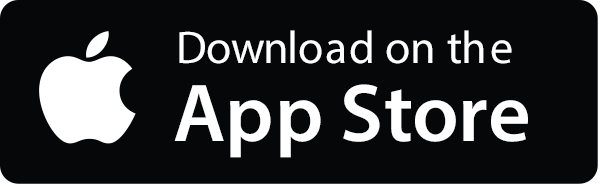
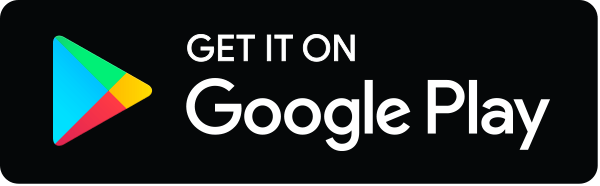
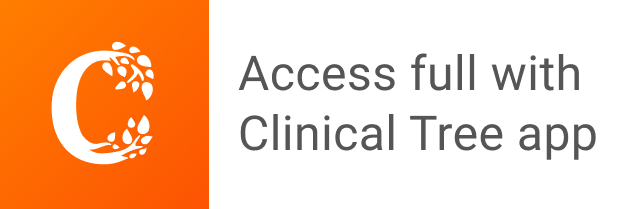