Historical Introduction to Vaccines
At the end of 2019, a novel human pathogen was first identified in China and resulted in a pandemic that posed the greatest infectious threat that humans had encountered in modern times. The virus responsible for the pandemic, severe acute respiratory syndrome coronavirus 2 (SARS-CoV-2), soon infected hundreds of millions of people, resulting in millions of deaths worldwide. To develop a critical understanding of the coronavirus disease 2019 (COVID-19) and its causative agent SARS-CoV-2, to study the evolution of the pandemic over time in different parts of the world, and eventually to control the pandemic, it is necessary to examine the challenges various viral infections posed over the centuries and the role of vaccines in containing them.
Smallpox became a scourge in the Indian subcontinent more than two millennia ago and gradually spread to other parts of the world. Although a vaccine was developed for the disease by Edward Jenner in the later part of the 18th century, the concept of virus evolved during the last decades of the 19th century and crystallized only in the early years of the 20th century. As nicely put by William Summers, viruses are a conceptualization of scientists. The “virus” described by Jenner in 1778 during smallpox vaccination, the view provided by Pasteur at the time of his rabies vaccine in 1885, and the “virus” concept provided by Stanley in 1935 when he crystallized the poliovirus are naturally different. The first human virus to be discovered was the yellow fever virus in 1901, and the first to be visualized was the tobacco mosaic virus in 1939 using an electron microscope. The first approach to combating viruses was by vaccination. Throughout the 19th and first half of the 20th century, all the vaccines were developed using viruses grown in animals and chicken egg embryos. This was the methodology for the development of vaccines for smallpox, rabies, yellow fever, and influenza. Polio represented an advance in our options for how we could manipulate and ultimately combat viruses; the successful growth of the poliovirus in cell cultures led to the development of the polio vaccine in the early 1950s of the 20th century using a cell culture approach.
Emerging viruses are defined as those causing new human infections that had never been encountered earlier, and reemerging viruses are defined as those causing opportunistic infections after lying quiescent for many years or even decades. Most emerging viruses are zoonotic, jumping to humans from wildlife reservoirs or through an intermediate domestic animal host. Human immunodeficiency virus (HIV), avian influenza viruses, Nipah virus (NiV), severe acute respiratory syndrome coronavirus (SARS-CoV), Middle East respiratory syndrome coronavirus (MERS-CoV), Lassa mammarenavirus, Zika virus, and the more recent severe acute respiratory syndrome coronavirus 2 (SARS-CoV-2) are prominent emerging viruses. The Ebolaviruses (Bundibugyo virus, Sudan virus, Tai Forest virus, and Ebola virus [formerly Zaire Ebola virus]) reemerged in the 1990s after the first well-recognized outbreak of the 1970s.
Viral evolution plays a significant role in the viral agent’s survival. By their mechanisms of mutation and recombination, the survival of many viruses is ensured, as variants arise that can evade the host immune responses. A classic case in point is the influenza A virus, which has not been eliminated even in the presence of effective vaccines and antiviral drugs with some efficacy such as amantadine and oseltamivir.
Viruses also have been found to be causative agents for many tumors. In 1911, Peyton Rous first demonstrated the transmission of sarcoma in chickens through cell-free filtrates. Since then, over the course of a century, many viruses, including human papillomaviruses (HPV), Epstein Barr virus, Kaposi sarcoma herpesvirus, human T-cell leukemia virus-1 and human T-cell leukemia virus-2, hepatitis B virus (HBV), and hepatitis C virus (HCV), have been implicated in various types of cancers, together causing a significant percentage of human cancer deaths. Vaccines also have been developed to prevent infection against some of these viruses, for example HPV and HBV.
Working with the fowl cholera bacterium, Louis Pasteur developed a method of growing and weakening the microbe in culture. He discovered that these weakened or attenuated bacteria caused a mild form of the disease and protected the chickens when exposed to the virulent form. Pasteur gave the name vaccine to this attenuated strain based on the Latin vacca which means cow. He was honoring Dr. Jenner and his work using cowpox inoculation to prevent smallpox.
The rest of the chapter is organized as follows. The chapter begins with a discussion of the science of vaccinology incorporating the basic concepts of the various types of immunity, and the different stages of the vaccine development process to ascertain safety and efficacy. The discussion then proceeds to describe the promising SARS-CoV-2 vaccines under various stages of development, with a focus on those undergoing phase III clinical trials, including those that have been accorded emergency use authorization (EUA) or approval by different regulatory agencies. Finally, the chapter provides a summary of the vaccine rollout under way in the developed and developing countries and the challenges posed by emerging SARS-CoV-2 variants for acquiring population immunity.
Vaccines and Vaccinology
The science of vaccinology is founded on the basic principles of immunology, which are outlined in the following section.
Innate and Adaptive Immunity
The human body fights infections using two basic immune mechanisms called innate and adaptive immunity. Innate immunity is the body’s first line of defense and is activated immediately within minutes to a few hours of the entry of pathogens. Macrophages and neutrophils are recruited to quickly engulf and destroy extracellular pathogens by phagocytosis. Pathogen recognition is achieved in an antigen-specific manner using related molecular structures called pathogen-associated molecular patterns (PAMPs). PAMPs are broad patterns made up of essential polysaccharides and polynucleotides found in various pathogens, but these are absent in the host receptors. In innate immunity there is no memory trigger of past exposures. “Natural antibodies,” which are evolutionarily selected immunoglobulins based mostly on germline sequences, also play a role in innate immune mechanisms.
Adaptive immunity is a tailored, precise, and specific response to pathogen entry. The body mounts an initial adaptive immune response over a period of a few days to weeks. The pathogen is recognized precisely by receptors that are generated by a random process of differentiation of B and T lymphocytes. Clones of highly specific T lymphocytes that can discriminate between even very small differences in the molecular structure of pathogen epitopes are generated and recruited for the adaptive immune response. The pathogen epitopes are made of polypeptides or polysaccharides and represent the uniqueness of a pathogen. A primary adaptive B-cell response takes about a week to be detected, but the memory of the event is retained and a secondary response typically has a shorter lag phase of 3 or 4 days. Moreover, in the primary response, low-affinity immunoglobulin M (IgM) antibodies predominate initially followed by the development of higher affinity IgG antibodies. High-affinity IgG antibodies dominate the secondary response. The kinetics of the initiation of an adaptive T-cell response follows a similar time course with the ability to detect expansion of T cells, with a T-cell receptor repertoire being first detectable 3 to 4 days after pathogen challenge. Fig. 18.1 presents a depiction of the primary and secondary immune response curves. Fig. 18.2 shows the interaction of innate and adaptive immune systems. The dendritic cells act as intermediaries between the innate and adaptive immune systems. The interested reader is referred to publications by Punt et al. and Virella for further details.
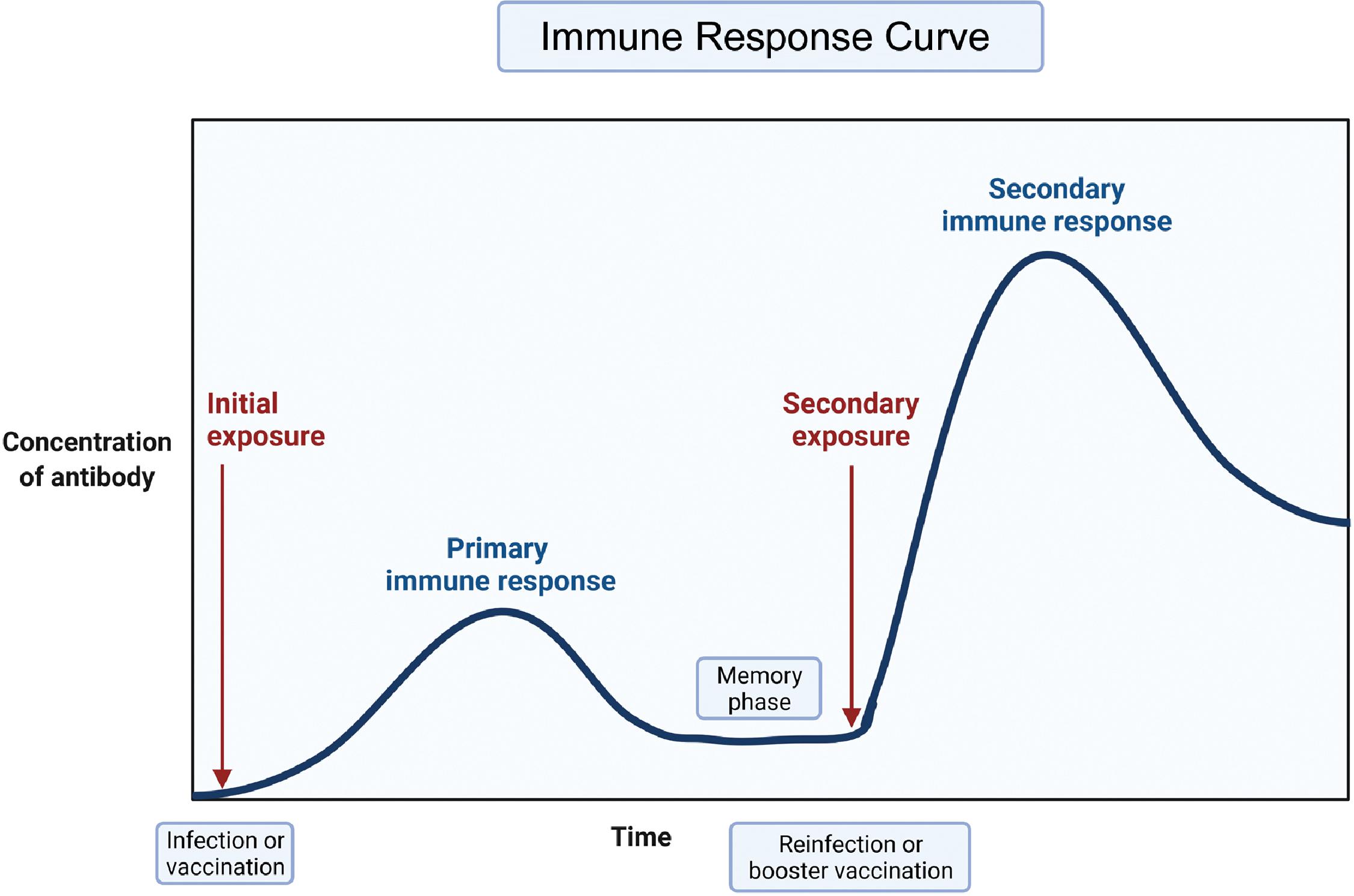
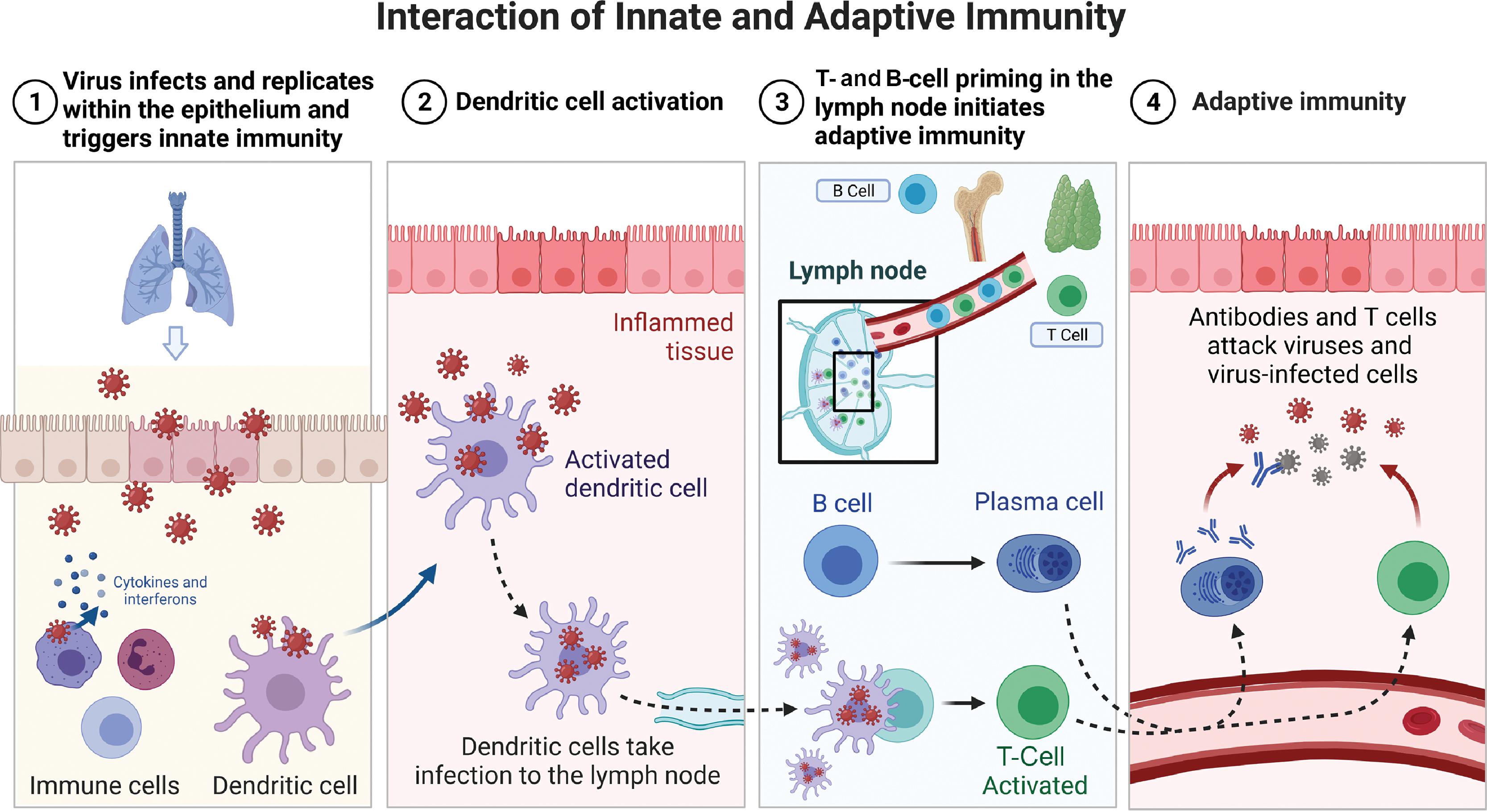
Population Immunity
In vaccinology, the concept of population immunity, also referred to as herd immunity, a
a Because herd pertains to nonhuman primates and animals, we prefer to use population immunity.
plays a significant role. When a community attains population immunity, it can be expected to be protected from the onslaught of a specific pathogenic infection. One way to obtain this type of immunity is by the natural course of disease transmission when a significant proportion (60%–95%) of individuals in a community are exposed and subsequently get infected by the pathogen. However, this can impose a high burden of mortality and morbidity on the population. A much more desirable approach to attain population immunity is through vaccination or immunization. However, the proportion of a population in a community or geographic region who must be immunized with a vaccine for a specific infectious disease depends on the transmissibility of the pathogen in question. For example, measles is a highly contagious disease, and for effective protection, 95% coverage is needed. This is to ensure that the entire population is shielded from the pathogen. Population immunity confers a direct protective effect on the immunized, but it also provides indirect protection for the nonimmunized individuals by disabling the transmission chain, as shown in Fig. 18.3 .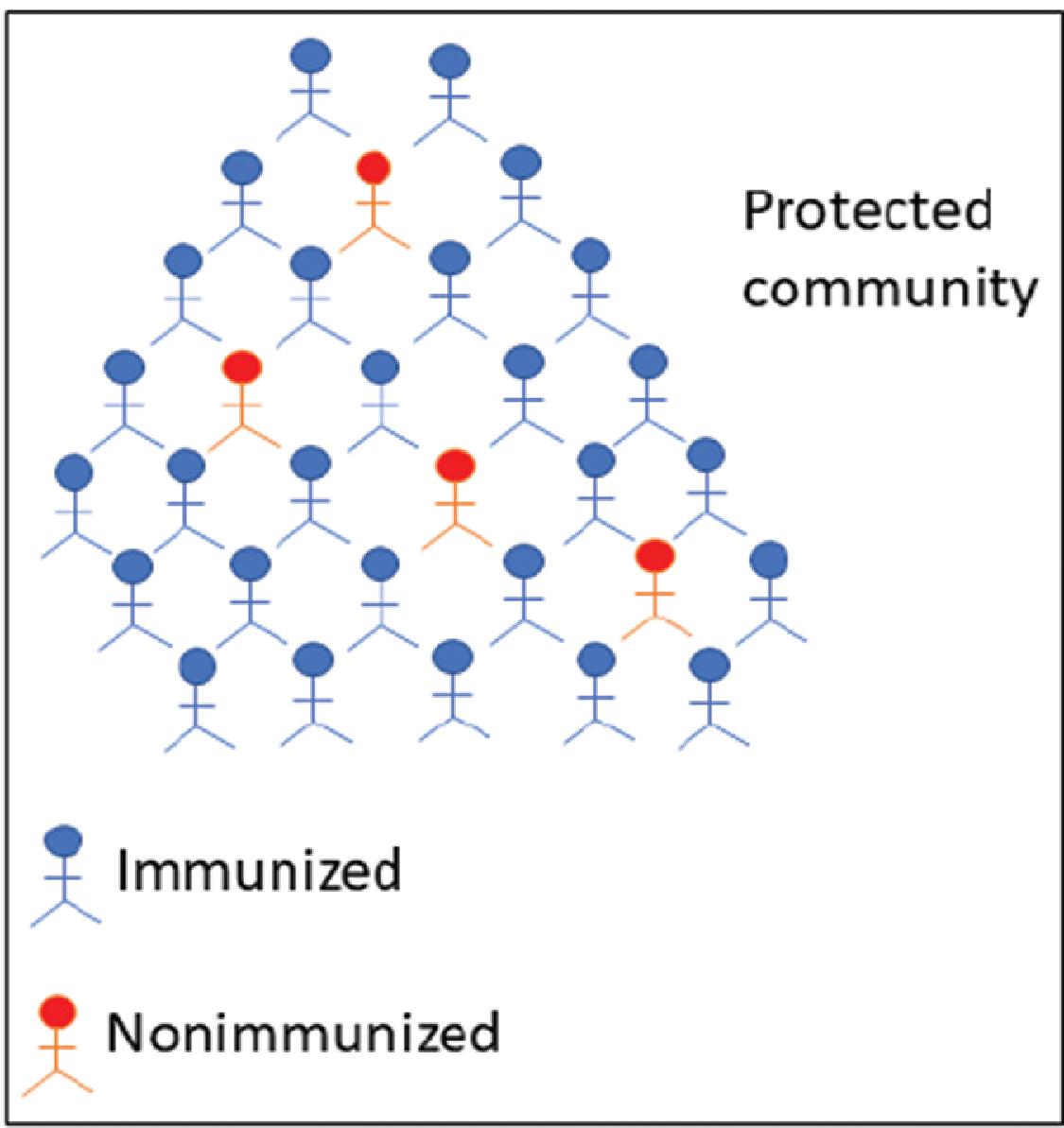
History of Vaccines
From a functional perspective there are two predominant categories of vaccines: prophylactic and therapeutic. Prophylactic vaccines are typically administered as part of a preventive strategy before a person is exposed to a pathogen or develops the disease. Therapeutic vaccines are administered as a treatment strategy for an existing disease or clinical condition.
Edward Jenner is credited with developing an approach to smallpox vaccination in 1796 using anecdotal evidence and previous observations that milkmaids who developed cowpox lesions were protected from smallpox. Although Jenner is properly credited for his investigations, publications, and prolific correspondence that championed vaccination, Benjamin Jesty, a farmer, had also become aware of the protection from smallpox that an earlier cowpox infection provided. Based on this knowledge, Jesty inoculated his wife and two sons with fluid from a cowpox lesion on one of his cows in 1774. This ultimately turned out to be a safer approach than the Turkish variolation method that involved introducing a small quantity of infective smallpox material into a skin incision, with a resultant 2% mortality. The credit for sheep anthrax vaccine goes to Louis Pasteur, who made a public demonstration of his anthrax vaccination methodology in 1881 by inoculating 25 sheep, with another 25 sheep retained as controls. When challenged with anthrax spores, 24 of the 25 vaccinated sheep survived compared with only 2 of 25 survivors in the control group. However, Pasteur did not give credit to Henri Toussaint, who developed the method to inactivate anthrax bacteria using phenol. Later, Pasteur developed a killed vaccine for rabies from air-dried spinal cords of infected rabbits. He successfully vaccinated a 9-year-old boy, Joseph Meister, bitten by a rabid dog in 1885, and Meister survived. This was the first therapeutic vaccination, because Meister was vaccinated after exposure to rabies.
Starting with serendipity and observation in the late 1700s, vaccine methodologies evolved into empiric approaches involving isolation, inactivation, and the use of killed microbes in the late 19th and first half of the 20th centuries. For diphtheria and tetanus, toxins were identified as the cause of the disease, and antiserum made against toxins in horses was used in passive vaccination. From 1880 to 1950, vaccines were developed successfully to tackle rabies, diphtheria, tetanus, and polio. The bacillus Calmette-Guérin (BCG) vaccine for tuberculosis was also developed during this period.
The second half of the 20th century saw two new successful vaccine development approaches: recombinant DNA technology and glycoconjugation or polysaccharide-protein conjugation. By 1980, the recombinant method yielded two effective vaccines, one for HBV and the other for HPV. By 1990, the polysaccharide-protein conjugation method enabled the development of the meningococcal ACWY (MenACWY), Haemophilus influenzae type b (Hib), and pneumonia vaccines. These newer vaccines are also referred to as subunit vaccines because they contain only parts of the pathogen but are capable of generating an adequate immune response after vaccination. In the first decade of the 21st century, genome-derived reverse vaccinology approaches using bioinformatics methods were employed to develop the meningococcal B (MenB) vaccine. Fig. 18.4 illustrates the vaccination milestones.
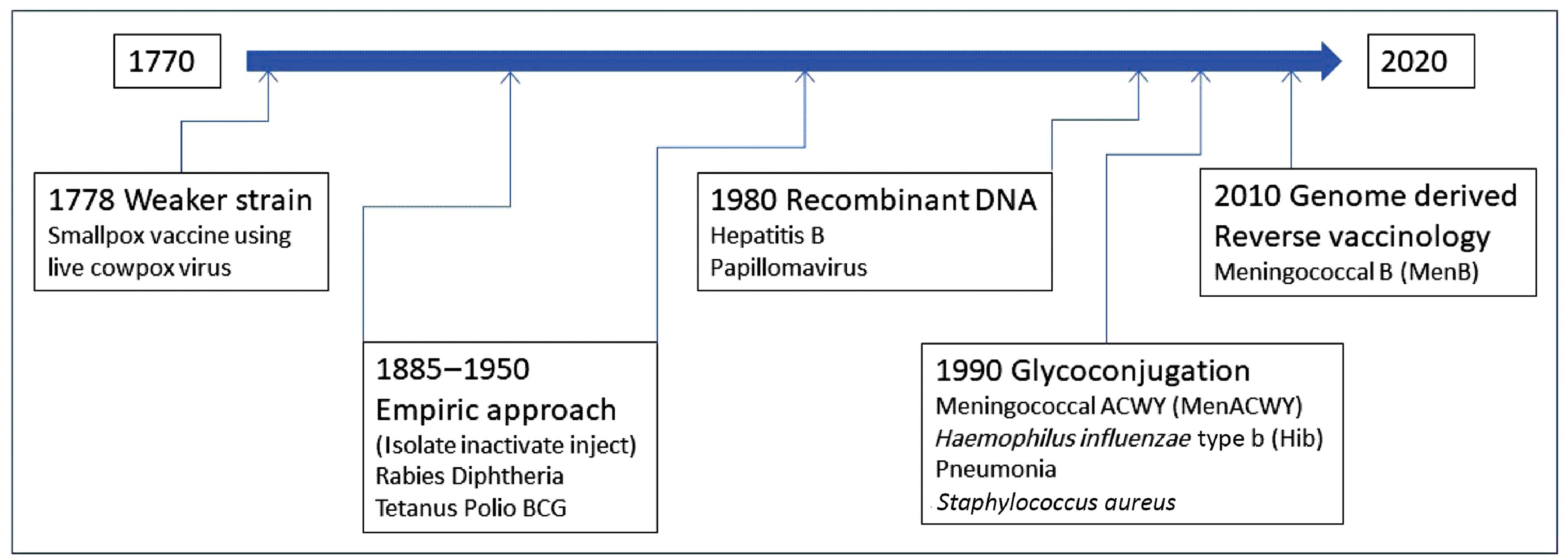
Public Health Impact of Vaccines
The 20th century saw the development of new and effective vaccines for many infectious diseases, resulting in a dramatic reduction in mortality and morbidity from the onslaught of these diseases. Just in the 20th century alone, smallpox killed more than 500 million people before the worldwide eradication strategy of the disease succeeded, and smallpox was eliminated as a disease in 1980. Although effective vaccines have been developed for influenza, approximately 30,000 people on average succumb to the complications resulting from influenza in the United States alone each year. This translates to approximately half a million deaths globally every year from influenza. Measles causes more than 100,000 deaths worldwide in spite of the availability of an effective vaccine from the 1960s. The reasons for the failure to control outbreaks of diseases in many countries of the world, even when effective vaccines are available, can be traced to economic disparities, widespread ignorance among the populace, lack of political will in the countries’ leadership, and inadequate allocation of resources for public health. However, a substantial increase in the life expectancy of children and by extension in the whole populace was observed globally in the second half of the 20th century as a result of the impact of vaccination and immunization campaigns led by the World Health Organization (WHO) and the respective public health authorities of various countries.
The dramatic reduction in mortality and morbidity of various infectious diseases observed in the last century in the United States clearly illustrates the significant impact of vaccines in the prevention and control of various infectious diseases. By the end of the 20th century, the percentage reduction in morbidity for smallpox, diphtheria, pertussis, tetanus, paralytic polio, measles, mumps, and rubella varied between 99.3% and 100% (see Table 18.1 for additional details). Paralytic polio is on the verge of global eradication, having been eliminated from the developed world and most developing countries. However, there were isolated reports of paralytic polio during a period of 19 months (January 2019 to July 2020) in 5 countries of Asia (Afghanistan, China, Myanmar, Pakistan, Philippines) and 14 countries of Africa. Among these countries, only Afghanistan and Pakistan have documented paralytic polio caused by wild-type poliovirus. Paralytic polio cases in the other countries are oral polio vaccine–associated paralytic poliomyelitis resulting from circulating vaccine-derived poliovirus.
Disease | 20th-Century Morbidity a | 1998 Morbidity b | Percent Decrease |
---|---|---|---|
Smallpox | 48,164 | 0 | 100 |
Diphtheria | 175,885 | 1 | 99.99 |
Pertussis | 147,271 | 6279 | 95.7 |
Tetanus | 1314 | 34 | 97.4 |
Polio (paralytic) | 16,316 | 0 | 100 |
Measles | 503,282 | 89 | 99.99 |
Mumps | 152,209 | 606 | 99.6 |
Rubella | 47,745 | 345 | 99.3 |
a Average annual number of cases before universal vaccine use recommended. Smallpox, 1900–1904; diphtheria, 1920–1922; pertussis, 1922–1925; tetanus, 1922–1926; polio, 1951–1954; measles, 1958–1962; mumps, 1968; rubella, 1966–1968.
b Number of cases reported for each disease in 1998. Modified from the Centers for Disease Control and Prevention. Ten great public health achievements—United States, 1900–1999. MMWR Morb Mortal Wkly Rep . 1999;48(12):241–243.
Types of Vaccines
As noted earlier, the field of vaccinology started with live attenuated and inactivated vaccines. During the last part of the 20th century, and more so in this century, the focus has shifted to subunit vaccines particularly for tackling emergent viral diseases. These subunit vaccines typically consist of surface proteins that can induce a significant immune response, and they could also be conjugated with polysaccharides. Subunit vaccines also can be engineered with DNA or RNA segments that encode for the immunogenic proteins. These vaccines are also referred to as recombinant vaccines. Because the recombinant vaccines consist of specific immunogens, they typically elicit a better immune response than inactivated vaccines. Recombinant vaccines are also considered safe when compared with live attenuated vaccines because there is no danger of virulence. The vaccine types are illustrated in Fig. 18.5 .
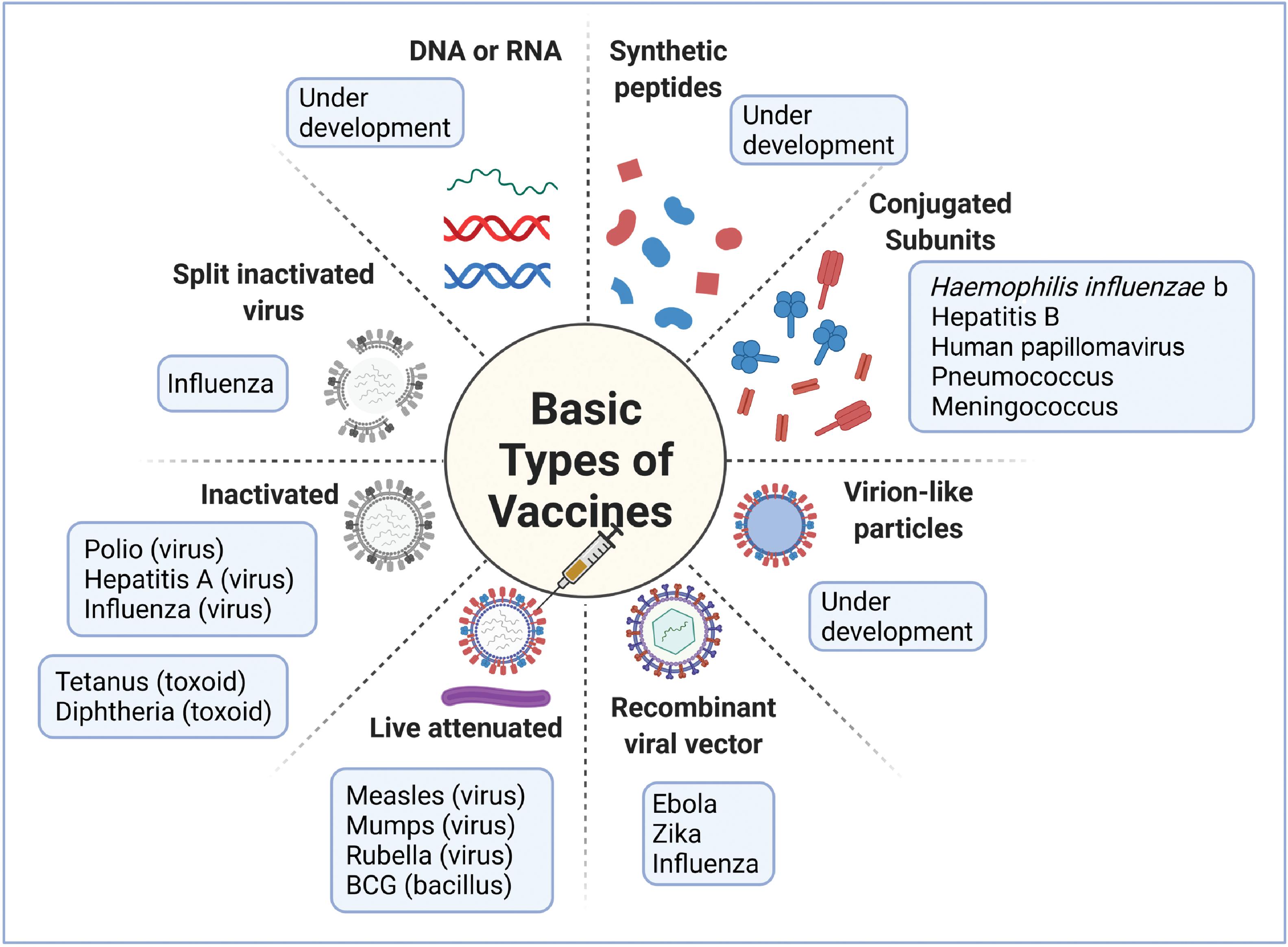
Nucleic Acid Vaccines
These vaccines use antigen-encoding plasmid DNA or RNA. In this case the vaccine does not possess the genome to make the microbe but just the genetic material necessary to make the required immunogens needed for protective immunity. The DNA has to be delivered inside cells and into the nucleus so that it can be transcribed first to messenger RNA (mRNA) and then translated into the relevant immunogenic proteins, making use of the cell machinery. The cells subsequently secrete the immunogens or they get attached to the surface. If mRNA is used, it need only enter the cytoplasm of cells, where it is directly translated into proteins using the host cellular machinery. Because mRNAs can be directly translated into proteins using the cellular ribosomal apparatus, they have a superior protein (antigen) expression profile. Moreover, antigen-specific targeting is facilitated by the expression of specific antigens in antigen-presenting cells (APCs). The body’s own cells are responsible for manufacturing the vaccine using their own cellular architecture and components, resulting in protective immunity. Both humoral and cell-mediated immune responses are generated by this method.
There are a few additional advantages for nucleic acid vaccines. These vaccines are cell-free. No handling of live virus is needed, so a biosafety level 2 (BSL2) laboratory is not needed for vaccine development. Moreover, production can be speeded up because manufacturing is synthetic. The disadvantage is that mRNA is fragile and cold storage is needed to prevent degradation.
Therapeutic Vaccines
Therapeutic vaccination involves the administration of a vaccine to manage or treat a preexisting disease or clinical condition. The general goal here is to target noninfectious diseases such as cancer, Parkinson disease, Alzheimer disease, multiple sclerosis, arthritis, or conditions such as obesity, high blood pressure, and drug addictions. The challenge here is to critically understand the disease pathogenesis and pathological conditions and come up with suitable targets for vaccination. Note that in the case of noninfectious diseases, we are dealing with host proteins as opposed to pathogen-derived proteins as targets, and this can cause problems with immune targeting. The recognition of specific conformational forms in the pathological processes associated with the disease is a key step in the development of effective therapeutic vaccines. , Identification of circulating cytotoxic T cells specific to tumor antigens indicates that induction of an immunogenic response to mutated tumor host proteins is feasible.
Therapeutic vaccines are broadly categorized into cancer vaccines and noninfectious noncancer (NINC) vaccines. Current cancer vaccines include personalized tumor immunotherapy, prostate cancer vaccine, and the use of BCG vaccine for bladder cancer. NINC vaccines are an area of intense investigation, with vaccines for addiction, high blood pressure, and obesity, currently in exploratory stages (see Fig. 18.6 for more details.)
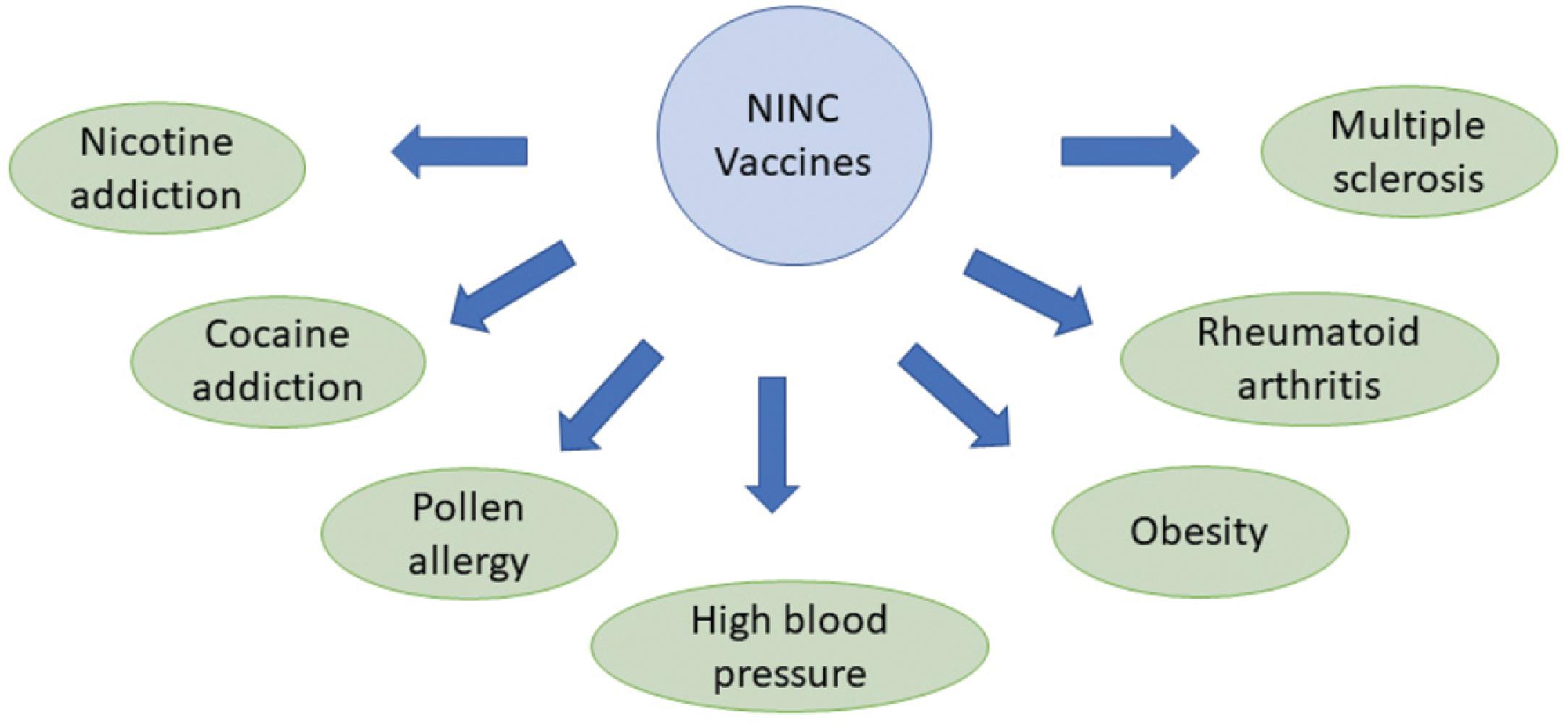
The first use of therapeutic vaccination was to address the infectious disease rabies. The vaccine is still in use effectively to this day for this purpose with excellent efficacy as long as it is used early enough after infection. There may be a role for therapeutic vaccination in patients with COVID-19 who continue to have symptoms past the initial 4 weeks of illness.
Vaccines, Immunity, and Age
Age exerts a significant influence on the immune system. Generating immunological response by vaccination is easier in the young, whereas immune protection is quite challenging in older people. Low efficacy of seasonal influenza vaccine in the elderly has been well documented. , Immune competence decreases with increasing age, which is termed immunosenescence. This can result from changes at multiple levels of the immune system over time, such as reduced lymphopoiesis in the bone marrow and thymus atrophy. These in turn result in a lower output of native T lymphocytes. Likewise, T-cell receptors and B-cell receptors are also negatively affected by aging. This implies that a conventional vaccine based on young adult responses may not be highly effective in older adults. , Supplementation of vaccination with immunotherapy by an infusion of neutralizing monoclonal antibodies might be needed in the elderly for enhanced protection.
Vaccine Protection Mechanisms
It is critical to understand what vaccines can and cannot do. Vaccines do not prevent pathogen entry or transient pathogen growth. Stimulating the host immune response by activating innate and adaptive immune mechanisms, vaccines can block pathogens from establishing a firm foothold by inhibiting the growth, development, and unchecked replication of these organisms. They can prevent the occurrence of clinical manifestations of disease or reduce the severity and complications resulting from infections. The goal of vaccination is to induce adaptive immunity that is specific for vaccine antigens. This facilitates the development of persistent antibodies, and memory B-cell and T-cell responses. This cascade of events protects the vaccinated individual during later contact with the virulent pathogen. The vaccine components engage the innate immune mechanism to induce protective adaptive immune responses.
Vaccine Development Process
Creating an effective and safe vaccine involves the successful completion of five critical stages: exploratory, preclinical, clinical trials, US Food and Drug Administration (FDA) review and approval, and, finally, manufacturing the required number of doses. These stages are usually sequential, and successful completion of a specific stage is necessary to move forward to the next stage. The COVID-19 pandemic has seen some changes to this paradigm with production often occurring before FDA review or approval. The exploratory stage incorporates in silico medicinal chemistry and biological experimentation, which leads to the preclinical stage, which typically involves testing for safety and efficacy on laboratory animals and possibility even primates. Successful completion of these two stages moves the process forward to the most important stage, the clinical trials stage, which will be discussed in some detail. Fig. 18.7 illustrates these five stages of the vaccine development process. The figure also shows the accelerated timeline with overlapping stages (stages 3, 4, and 5) for possible emergency use authorization in the case of pandemics.
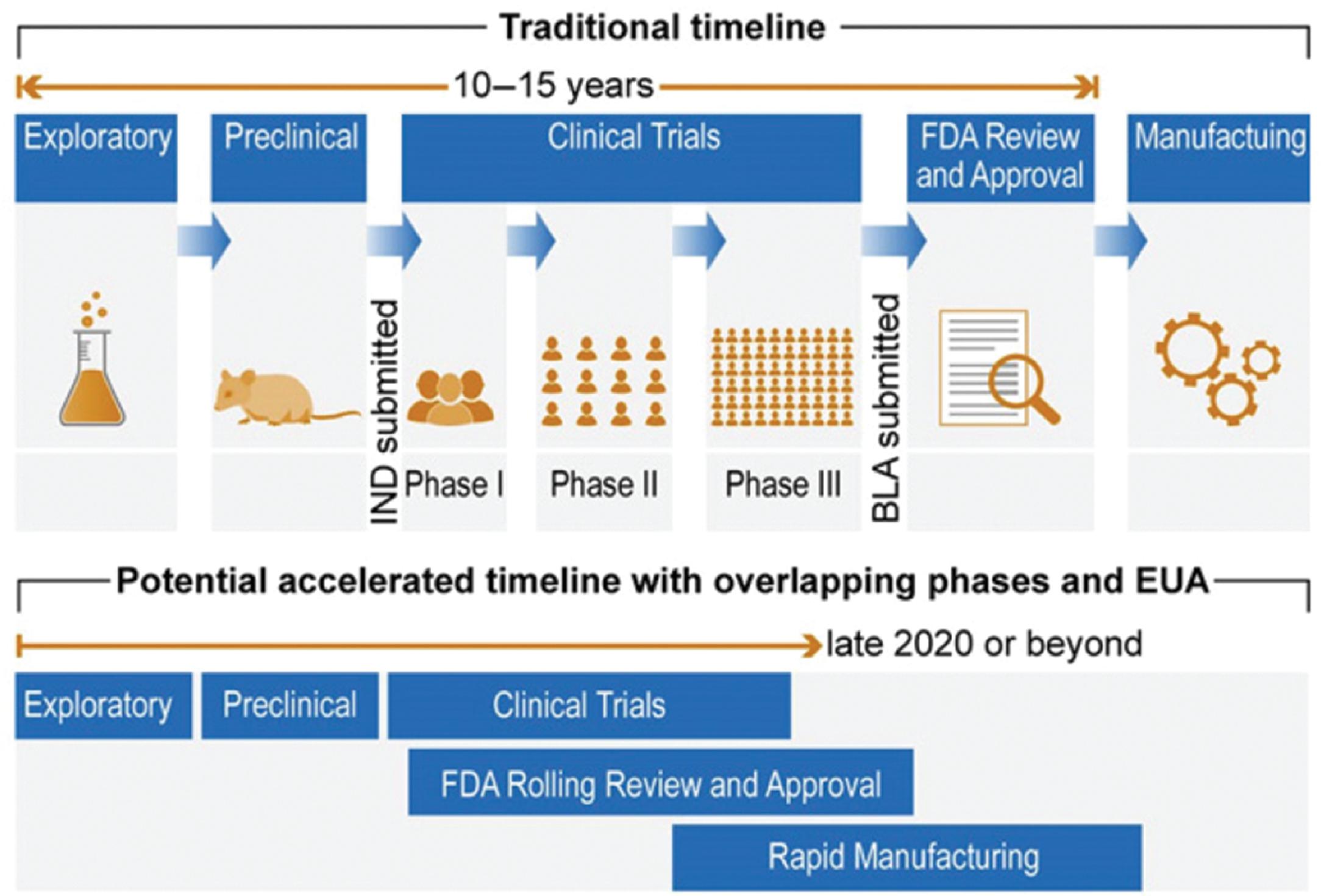
Clinical Trials
A candidate vaccine advances to the clinical trials stage to evaluate the safety and efficacy of the vaccine in a rigorous and transparent manner. This task is accomplished by progressively larger complex human trials that are conducted in three sequential phases: phases I, II, and III. Safety of the candidate vaccine is assessed using healthy volunteers in phase I. In phase II, preliminary efficacy, dosage protocol, and additional safety determinations are made. The phase III clinical trial is the most critical, complex, and challenging step of the vaccine development process. Typically, it is a large randomized, placebo-controlled, double-blinded clinical trial involving the enrollment of thousands or even tens of thousands of subjects based on strict inclusion and exclusion criteria. It is clearly an expensive and resource-intensive undertaking. Successful completion of this important phase is mandatory for regulatory review and approval; the vaccine then moves on to the manufacturing stage. Fig. 18.8 presents additional details of the different phases of the clinical trials stage for a COVID-19 candidate vaccine.
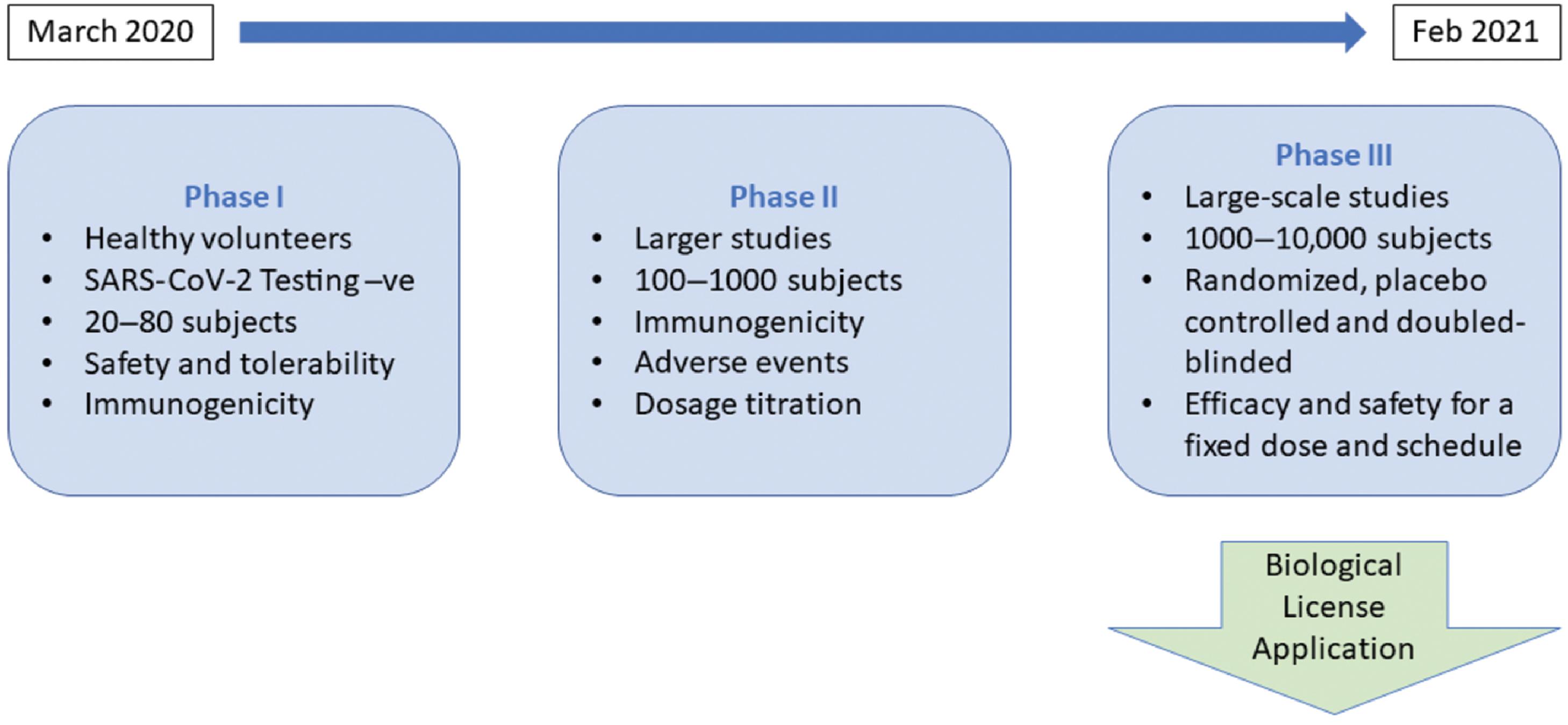
Vaccine Development Timeline
The vaccine development process involves five sequential stages, including a three-phase clinical trial stage; it usually takes many years to decades to develop a successful vaccine. For example, development of the meningococcal B vaccine, including licensing, took almost 15 years ( Fig. 18.9 ). The shortest recorded time was for the live attenuated Jeryl Lynn mumps vaccine, developed by Maurice Hilleman at Merck laboratories in a span of about 5 years.
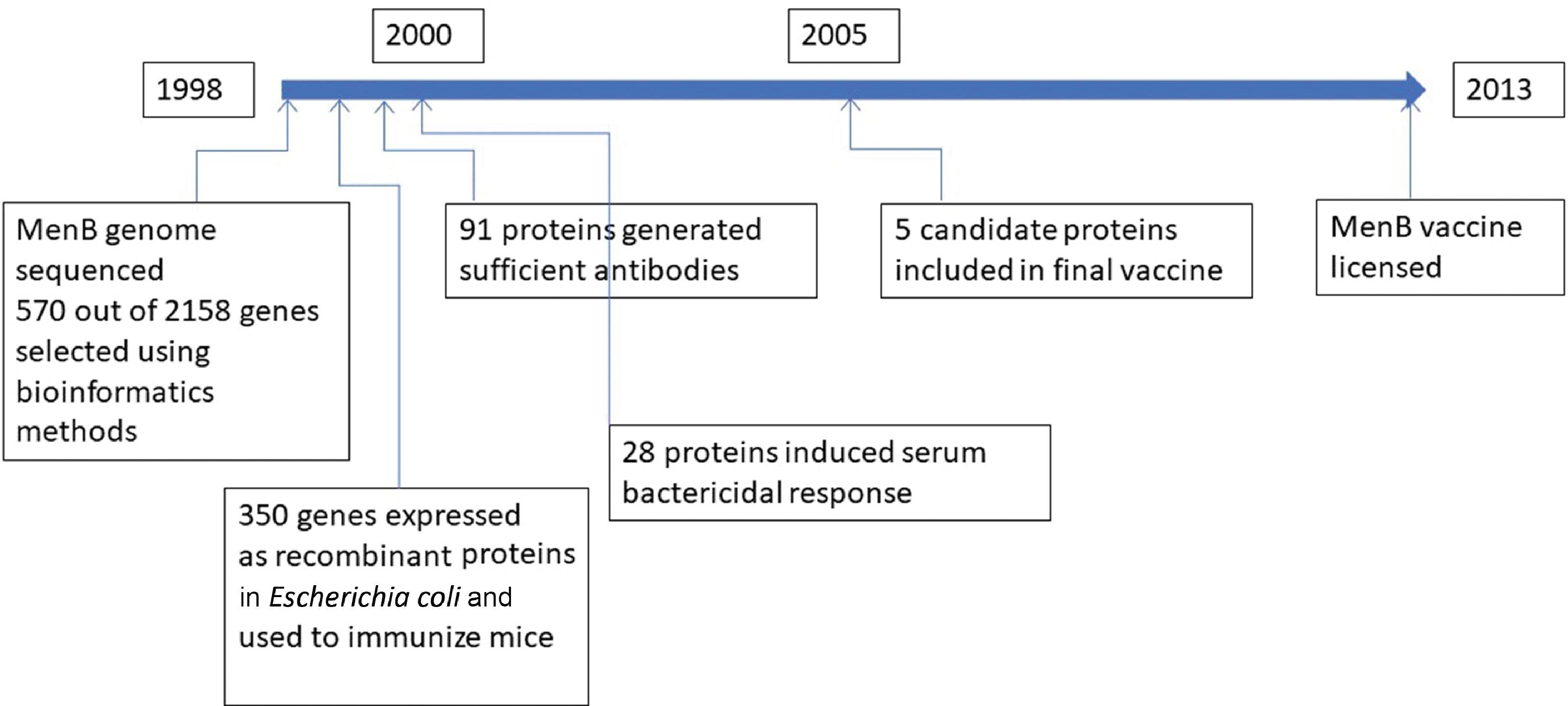
Vaccine Development Failures
Even when the causative pathogen is isolated and sequenced, a safe and effective vaccine may not materialize in spite of persistent efforts. For example, there are no licensed effective vaccines currently in the market for HIV, HCV, and malaria. HIV mutates continuously in the human host, which poses significant challenges for vaccine development. HCV shows enormous diversity, and animal models for testing potential vaccines are limited. Moreover, our understanding of protective immune responses against HCV are still evolving. In general, developing vaccines against parasites is problematic because of their larger genomes. The malarial parasite genome has more than 5000 genes, which provide hundreds of potential targets and a plethora of vaccine candidates over the different stages of the parasitic life cycle. We need a better understanding of the mechanisms and key targets of immunity to develop a successful and durable vaccine for malaria.
COVID-19 Vaccine Development
COVID-19 emerged as the biggest public health challenge mankind has faced over the last 100 years. To combat the disease and bring the pandemic under control, various teams, spread over many countries, are engaged in developing successful vaccines against SARS-CoV-2.
Vaccine Candidate Mechanisms
Apart from the more traditional approaches of using an attenuated and inactivated virus, many of the leading candidate vaccines use one of these three methods: mRNA coding a SARS-CoV-2 gene, recombinant SARS-CoV-2 surface protein, and viral vector packaging SARS-CoV-2 gene, as the key mechanism to induce an effective antibody response in the vaccinated host. These approaches are illustrated in Fig. 18.10 . The live attenuated, mRNA, recombinant surface protein and viral vector incorporating SARS-CoV-2 gene(s) approaches induce both humoral and cellular immunity–like natural infections. However, the inactivated pathogen-based vaccines can initiate only a humoral immune response.
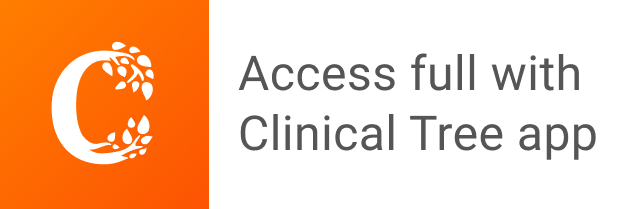