Fig. 9.1
Gross anatomy of the neck showing the thyroid gland in relation to nerves, vasculature, and muscles [81]
The thyroid gland consists of follicles, lined with a single layer of epithelial cells that actively transport iodine into the follicle as well as generate and secrete thyroid hormones, T3 and T4 (Fig. 9.2a, b). The follicles are filled with colloid, rich with the high-molecular-weight protein thyroglobulin necessary for thyroid hormone synthesis as well as the hormonally inactive monoido- and diiodo-tyrosines [2].
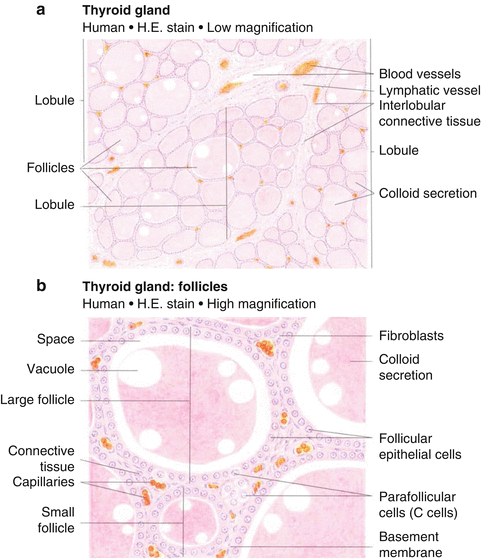
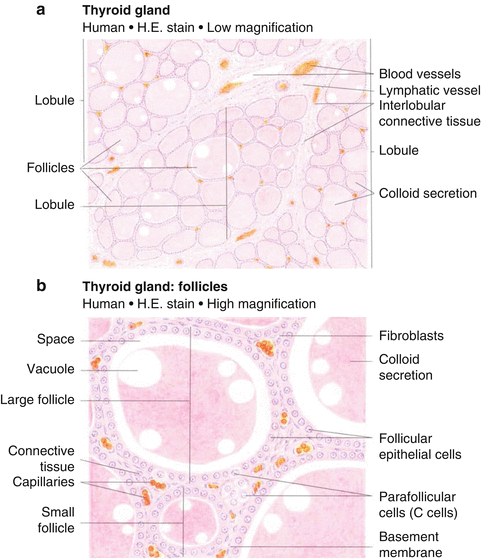
Fig. 9.2
Histologic appearance of the thyroid gland at low (a) and high (b) magnification. At high magnification, the follicular epithelial cells and parafollicular cells are readily apparent [82]
9.1.2 Thyroid Endocrine Dysfunction
Generally, radiation changes in the thyroid gland result from damage to small vessels (remote branches off of the superior and inferior thyroid arteries) and presumably less so from direct damage to the fully differentiated follicular epithelial cells. This relates to their slow proliferative rate that makes them relatively less sensitive to radiation injury [2, 3]. The endothelial cells of the thyroid gland vasculature may have proliferation cycles shorter than those of endocrine cells. Thus, Rubin and Cassarett postulated that the initial radiation injury to the thyroid gland was more specifically to the endothelium of small vessels, and with additional radiation exposure, the follicular epithelial cells degenerate as a result of further damage to the vasculature [1], a concept further supported by Fajardo [4].
Histopathologic changes in an irradiated thyroid gland include progressive obliteration of the fine vasculature, degeneration of follicular cells and follicles, and atrophy of the stroma [1]. Because radiation damage is dependent on the degree of the mitotic activity and because the thyroid of a developing child grows in parallel with the body, this gland might be expected to show an age-related degree of injury and repair. Late changes are primarily attributable to vascular damage, while acute and subacute changes may be more affected by repairable damage to the epithelioid cells [3]. Acute radiation changes to the thyroid include diminished follicle size with low cuboidal epithelium lining residual follicles. With high radiation doses, including therapeutic I131 exposure, there is follicular necrosis, vasculitis, thrombosis, and hemorrhage followed by vascular sclerosis and lymphocytic infiltration [2]. Chronic changes after relatively low-dose exposure include focal and irregular follicular hyperplasia, hyalinization, fibrosis beneath the vascular endothelium, lymphocytic infiltration, thyroiditis, cellular and nuclear atypia, infarction, and necrosis [2, 5].
9.1.3 Thyroid Nodules and Malignancy
Ionizing radiation can cause DNA damage that leads to somatic mutations. Double-strand breakage can result in genomic rearrangements, which can cause deactivation of tumor suppressor genes or activation of proto-oncogenes. This ultimately increases the risk of development of both benign and malignant tumors.
Investigators studying radiation-induced papillary thyroid cancer have indentified specific translocations in the RET gene which when altered in this manner is referred to as the RET/PTC oncogene; this translocation results in constitutive expression and activation. Molecular and biologic studies of pathologic specimens of papillary thyroid carcinoma from individuals exposed to radiation following the Chernobyl accident in 1986 have found an increased incidence in several isoforms of the RET/PTC rearrangements compared to those from papillary thyroid cancer specimens as a whole [6, 7]. The frequency of RET translocations also has been found to be increased in thyroid cancer cases associated with external radiation treatment for benign and malignant condition [7, 8].
Further support for this etiology of radiation-induced thyroid malignancy is provided by the observation that point mutations in the BRAF gene, a common genetic activation in papillary thyroid cancer in the general population, are rarely found in radiation-related cases [9]. Additionally, the role of radiation-related translocations is further supported by the occurrence of TRK oncogenes [10].
It is not entirely clear whether radiation-related papillary thyroid cancers containing translocations behave differently from cancers without them; however, one of the above noted studies did find that cancers with the RET/PTC rearrangements had more aggressive behavior than BRAF-positive tumors or PTC without gene alterations [6].
9.2 Clinical Manifestations
Hypothyroidism may manifest with classic symptoms, including weight gain, cold intolerance, dry skin, brittle hair, constipation, menstrual irregularities, muscle cramping, and slower mentation; classic signs include periorbital and peripheral edema, hypotension, bradycardia, pericardial effusions, pleural effusions, and prolonged relaxation of deep tendon reflexes. Hypothyroidism may be occult, detected only by low thyroxine levels and/or elevated TSH [11]. This is often referred to as subclinical or covert hypothyroidism, as opposed to clinical or overt hypothyroidism, in which the hypothyroidism is associated with clinical symptomatology. Compensated hypothyroidism occurs when the pituitary gland releases high levels of TSH and thus overworks the thyroid gland to maintain adequate levels of circulating thyroid hormones. With uncompensated hypothyroidism, T4 remains low despite elevated TSH. Radiation-induced occult hypothyroidism is likely to progress to clinical hypothyroidism [2], though TSH levels can spontaneously normalize over long time intervals [2, 12].
Hyperthyroidism can manifest with classic symptoms including palpitations, heat intolerance, anxiety, fatigue and insomnia, diarrhea, menstrual irregularities, weight loss, and weakness. Clinical signs include goiter, tachycardia, tremor, warm moist skin, thinner skin, fine hair and alopecia, and infiltrative ophthalmopathy. Grave’s disease is an autoimmune form of hyperthyroidism resulting from the production of antibodies which bind to and chronically stimulate the receptor for thyroid-stimulating hormone (expressed on the follicular cells of the thyroid gland) causing increased production of the T3 and T4 hormones.
Thyroid cancer usually presents as a solitary, asymptomatic nodule [13]. The vast majority of children and adults with thyroid nodules have normal thyroid function. Sparse data exist on the presenting characteristics of children with secondary thyroid cancer. However, it is worth noting that children with de novo thyroid cancer, especially those who are prepubescent, more often present with advanced disease as compared with adolescents and adults [13, 14]. A review of over 500 pediatric patients from nine large referral centers found that the great majority of cases presented with only an isolated neck mass. However, after careful physical examination, over half of patients had palpable lymph nodes. Postoperative pathology revealed microscopic regional lymph node involvement in 71–90 %, extracapsular extension with invasion of the trachea in 20–60 %, and involvement of the recurrent laryngeal nerve in 30 %. The most common site of distant metastases was the lung (10–28 %), followed by the bones and brain [13]. These and other data suggest that thyroid carcinoma in the pediatric age group is a biologically independent and more aggressive entity than in adults.
9.2.1 Hypothyroidism
9.2.1.1 Radiation for Childhood Malignancies
Because of the incidental radiation to the thyroid gland in the treatment of Hodgkin lymphoma, many studies have specifically addressed late thyroid complications in this group. The incidence of hypothyroidism noted following therapeutic irradiation for Hodgkin lymphoma varies, depending on the report [3]. If an elevated serum TSH concentration is the determinant, then 4–79 % of patients become affected. This large range exists because parameters relevant to the induction of hypothyroidism – such as radiation dose, technique, and the frequency and types of follow-up testing – differ in the various reports. Most studies show the incidence of hypothyroidism progressively rising in the first 3–5 years after radiation, with most patients manifesting clinical signs or symptoms 2–4 years after radiotherapy [15–17]. Some studies demonstrate additional cases of hypothyroidism beyond 5 years [15–18], and rare and unexpected new cases can occur more than 20 years after diagnosis of Hodgkin lymphoma [16, 17].
A classic study by Hancock and colleagues [16] of 1,677 children and adults with Hodgkin lymphoma in whom the thyroid had been irradiated showed that the actuarial risk at 26 years for overt or subclinical hypothyroidism was 47 % (Table 9.1), with the peak incidence occurring 2–3 years after treatment. There are several reports specifically addressing the incidence of hypothyroidism in children as a function of radiation dose (Table 9.2) [12, 16, 17, 19–21]. Constine and colleagues [12] noted thyroid abnormalities in 4 of 24 children (17 %) who received mantle irradiation of 26 Gy or less and in 74 of 95 children (78 %) who received greater than 26 Gy. The abnormality in all but three children (one with hyperthyroidism and two with thyroid nodules) included an elevated serum TSH concentration with or without low serum T4 concentration. The spontaneous return of TSH to normal limits was observed in 20 of the 75 patients (27 %).
Table 9.1
Thyroid disease after treatment of Hodgkin’s disease
Disease | No. of patients/total no.a | Actuarial risk (%) | Time to occurrence (year) | ||
---|---|---|---|---|---|
20 year | 26 years | Median | Range | ||
At least one thyroid disease | 573/1,787//> | 50 | 63 | 4.6 | 0.2–25.6 |
570/1,677//> | 52 | 67 | 4.6 | 0.2–25.6 | |
Hypothyroidism | 513/1,787//> | 41 | 44 | 4.0 | 0.2–23.7 |
512/1,677//> | 43 | 47 | 4.0 | 0.2–23.7 | |
Graves’ diseaseb | 34/1,787//> | 3.1 | 3.1 | 4.8 | 0.1–17.6 |
32/1,677//> | 3.3 | 3.3 | 4.9 | 0.1–17.6 | |
Graves’ ophthalmopathyb | 21/1,677//> | – | – | – | – |
Silent thyroiditis | 6/1,677//> | 1.6 | 0.6 | 3.7 | 0.8–15.3 |
Hashimoto’s thyroiditis | 4/1,677//> | 0.7 | 0.7 | 7.9 | 3.5–15.2 |
Thyroidectomy | 26/1,677//> | 6.6 | 26.6 | 14.0 | 1.5–25.6 |
Thyroid cancer | 6/1,677//> | 1.7 | 1.7 | 13.3 | 9.0–18.9 |
Benign adenoma | 10/1,677//> | – | – | 12.0 | 1.5–25.6 |
Adenomatous nodule | 6/1,677//> | – | – | 17.4 | 12.7–24.4 |
Multinodular goiter | 4/1,677//> | – | – | 14.8 | 10.8–19.4 |
Clinically benign nodule | 12/1,677//> | 3.3 | 5.1 | 12.6 | 2.4–22.6 |
Clinically benign cyst | 4/1,677//> | 0.7 | 0.7 | 8.1 | 1.6–16.7 |
Multinodular goiterc | 2/1,677//> | 0.5 | 0.5 | 13.8 | 10.5–17 |
Table 9.2
Radiation dose to the thyroid gland and the risk of hypothyroidism
Institution | Patient population | # | Risk of hypothyroidism |
---|---|---|---|
Stanford U [12] | Children with Hodgkin lymphoma | 119 | Thyroid disorders (mostly hypothyroidism): 78 % for >26 Gy versus 17 % for ≤26 Gy |
Stanford U [16] | Adults and children with Hodgkin lymphoma | 1,787 | Clinical hypothyroidism: 20 % for ≥30 Gy versus 5 % for 7.5–30 Gy at 20 years, p = 0.001 |
Subclinical or clinical hypothyroidism: 44 % for ≥30 Gy versus 27 % for 7.5–30 Gy at 20 years, p = 0.008 | |||
Childhood Cancer Survivor Study [17] | Children with Hodgkin’s disease | 1,791 | Patient reports “underactive thyroid” 50 % for ≥45 Gy versus 30 % for 35–45 Gy versus ~20 % for <35 Gy at 15 years |
Joint Center for Radiation Therapy [19] | Childhood cancer | 95 | Elevated TSH: 68 % for ≥30 Gy versus 15 % for <30 Gy p = 0.007 |
RiSK Study [20] | Childhood cancer | 404 | Elevated TSH versus prophylactic cranial RT |
15–25 Gy to thyroid gland, RR 3.1 (p = 0.002) | |||
>25 Gy to thyroid gland, RR 3.8 (p = 0.009) | |||
Cranio-spinal radiation, RR 5.7 (p < 0.001) | |||
Turkey [21] | Children with Hodgkin’s disease | 55 | Abnormal thyroid function: |
27 % (6 of 22) after 25.2 Gy | |||
7 % (1 of 14) after 30.6 Gy | |||
83 % (5 of 6) after 36 Gy |
A report by Sklar [17] of 1,791 patients from the Childhood Cancer Survivor Study (CCSS) cohort showed that the relative risk of hypothyroidism in Hodgkin lymphoma survivors was 17.1, with 28 % of the cohort affected. The average time to developing hypothyroidism was 5 years. In patients who were treated with chemotherapy alone, the incidence of hypothyroidism was 7.6 %. In a multivariate analysis, the major risk factors associated with the development of hypothyroidism were radiation dose, female sex, and older age at diagnosis. The actuarial risk of developing hypothyroidism 20 years after a diagnosis of Hodgkin lymphoma was 30 % for patients whose thyroid received 35≤45 Gy and 50 % for patients whose thyroid received ≥45 Gy. Elapsed time since diagnosis was a risk factor, where the risk increased in the first 3–5 years after diagnosis [17]. Figure 9.3 shows, from this study, the time dependence of hypothyroidism, grouped by radiation dose to the thyroid [17].
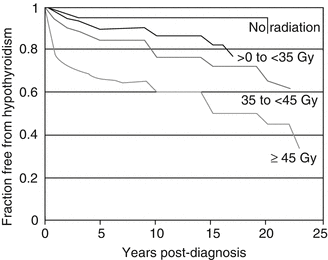
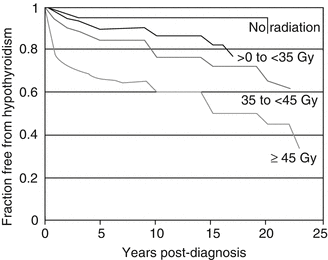
Fig. 9.3
Dose dependence of hypothyroidism risk from a study of 1,791 survivors of childhood cancer (Data and figure was derived from reference [17], in which actuarial rates of hypothyroidism were calculated among 377 patients who received no radiotherapy and 1,210 patients for whom sufficient data were available to estimate the dose of radiation to the thyroid gland (median 35 Gy and range 0.004–55 Gy))
The cumulative incidence of hypothyroidism among survivors 15 years following leukemia diagnosis has also been evaluated in the CCSS and was 1.6 %. In multivariate analysis, survivors who received ≥20 Gy cranial radiotherapy plus any spinal radiotherapy had the highest risk of subsequent hypothyroidism (hazard ratio 8.3) compared with those treated with chemotherapy alone. In radiation dosimetry analysis, pituitary doses ≥20 Gy combined with thyroid doses ≥10 Gy were associated with hypothyroidism [22]. In another study of 95 patients with childhood cancer [19] elevated TSH 5–34 years after radiation was seen in 68 % of patients who received ≥30 Gy to the thyroid versus 15 % of patients who received <30 Gy (p = 0.007). In the recent RiSK study of 404 childhood cancer survivors [20], compared to those who received prophylactic cranial RT (median dose 12 Gy), the relative risk of elevated TSH was 3.1 (p = 0.002) and 3.8 (p = 0.009) after doses of 15–25 Gy and >25 Gy to the thyroid gland, respectively; the relative risk was 5.7 (p < 0.001) for those who underwent cranio-spinal radiation.
Uncompensated hypothyroidism (decreased serum T4 and elevated serum TSH concentration) occurs in 6–27 % of children receiving radiation to the thyroid. In the study by Constine and colleagues, age did not affect the incidence of hypothyroidism but was weakly correlated with the degree of abnormality, as suggested by higher serum TSH concentrations in adolescents compared with younger children [12]. In reports from Hancock [16], Sklar [17], and Constine [12] older age at treatment of Hodgkin lymphoma was a major risk factor for future development of an underactive thyroid. This may reflect the greater sensitivity of the thyroid gland in rapidly growing pubertal children, compared with preadolescents, or it may reflect the fact that the older children generally received a higher radiation dose than the younger children.
9.2.1.2 Influence of Chemotherapy
The role of chemotherapy in producing thyroid abnormalities is less understood. The influence of chemotherapy on the development of thyroid dysfunction among Hodgkin patients appears to be negligible in most reports [12, 17–19, 21, 23, 24], although some data of patients with brain tumors (most of whom received cranial-spinal radiation) suggest that chemotherapy may add to the frequency of compensated hypothyroidism [25]. Notably, the thyroid gland in many chemotherapy-treated brain tumor patients (i.e., medulloblastoma) receives higher doses of radiation than in non-chemotherapy-treated patients.
9.2.1.3 Stem Cell Transplantation
Patients undergoing a bone-marrow transplant who receive total body irradiation (TBI) are also at risk for thyroid injury due to direct injury to the thyroid gland. Sklar [26] found that a single dose of 7.5 Gy caused a decrease in serum T4 concentration in 9 % patients and an elevated serum TSH concentration in 35 %. The frequency of overt hypothyroidism following transplantation is highly variable and depends largely on the conditioning regimen. Hypothyroidism after a 10 Gy, single dose of TBI can occur in up to 90 % of patients [27] versus 14–15 % in patients treated with fractionated TBI [28, 29]. Previously, regimens that did not involve whole-body irradiation were not associated with an increased risk, or at least a smaller risk [30]. However, in a report from the Fred Hutchinson Cancer Research Center, the increased risk of thyroid dysfunction was not different between children receiving cyclophosphamide and whole-body irradiation and those receiving a busulfan-based chemotherapy regimen (p = 0.48), while those who received cyclophosphamide conditioning alone experienced significantly lower risks (HR 0.32, p = 0.03) The highest risk of thyroid dysfunction was in the cohort treated with both busulfan and TBI (HR 1.7, p = 0.009) Although these data suggest that both TBI and busulfan may contribute to risk for thyroid dysfunction, the magnitude of the risk associated with the chemotherapy-only treatment is likely confounded by clinical selection of chemotherapy only for younger children [31].
9.2.2 Hyperthyroidism
Hyperthyroidism occurs less commonly after radiation than does hypothyroidism. Thirty of 1,787 (1.7 %) Hodgkin lymphoma patients (1,677 treated with radiation) at Stanford developed hyperthyroidism at a mean of 5.3 years (0–18 years) post treatment [16]. Ten had previously been treated for hypothyroidism. In addition, four clinically hypo- or euthyroid patients developed infiltrative ophthalmopathy consistent with Grave’s disease. Overall, this study showed a 7.2–20.4-fold increased risk of Grave’s disease [16]. In the CCSS cohort, including 1,791 patients with childhood Hodgkin lymphoma, hyperthyroidism developed in 4.5 % (RR = 8) at 0–22 (mean of 8) years after diagnosis [17]. Significantly increased risk was associated with doses ≥35 Gy.
Because hyperthyroidism and thyroiditis are much less common late effects after radiation than hypothyroidism, the risk factors associated with these complications are not well documented. Univariate analysis did not find gender, radiation dose, or chemotherapy exposure to be significant risk factors for hyperthyroidism [16]. An immunologic basis has been hypothesized based on the higher frequency noted in Hodgkin lymphoma patients versus others treated with incidental neck irradiation and is consistent with finding of a Hungarian study that noted a fourfold increase in the prevalence of serum antithyroglobulin antibodies in irradiated females with Hodgkin lymphoma [18]. Similarly, investigators at Roswell Park noted that the addition of chemotherapy to radiation significantly reduced the risk of thyroid autoantibodies (to 17 % versus 48 %, p < 0.01), bringing the baseline prevalence to that seen in the general population [15].
9.2.3 Thyroid Nodules and Malignancy
Thyroid radiation results in an increased risk of benign adenoma formation as well as thyroid cancer. When evaluating the incidence of thyroid nodules after radiation therapy, it is important to note the high baseline frequency of asymptomatic thyroid nodules in the general population: upon careful physical exam, clinically palpable nodules are found in 4–7 % of normal adults and in as many as 35–50 % of pathologic specimens following surgery or autopsy [32].
In a series of 87 patients treated with radiation to the neck for childhood cancer at the Joint Center for Radiation Therapy, 32 developed palpable thyroid abnormalities including nodules (n = 25) and diffuse enlargement (n = 7) [19]. The risk of palpable abnormalities was independent of radiation dose and more commonly seen in patients with longer follow-up, who were more likely to have received orthovoltage radiation. Resections in 10 of the 32 patients revealed hyperplastic follicular adenomas (n = 8), papillary thyroid cancer (n = 3), and an atypical malignant schwannoma (n = 1).
Roughly one-tenth to one-third of thyroid nodules developing in adult patients years to decades after radiation after childhood radiation proved to be malignant [17, 19, 33, 34]. This translates into a greater than 10 to 50-fold increased risk of thyroid cancer [17, 35–42]. In a study of 5,379 patients treated with radiation for benign conditions of the head and neck, incidences for thyroid nodules were 6 % for malignant and 8–12 % for benign lesions after low-dose (<15 Gy) external beam radiation [43]. Generally, radiation-induced thyroid malignancies are localized to the thyroid or regional lymph nodes and readily curable.
Historically, low-dose radiation (as low as 0.1 Gy) for benign disease (such as tinea capitis, thymic enlargement, lymphoid hyperplasia, lice, acne) has been a well-established risk factor for thyroid nodules and malignancy, with higher dose [44–46] and younger age at exposure associated with increased risk [46]. Overall, in this setting (low-dose radiation, generally 2–5 Gy, for benign conditions) new nodules develop at a rate about 2 % per year, with a peak incidence at 15–25 years [47].
In the 1970s–1980s, case reports were published suggesting that the higher therapeutic radiation doses used to treat malignancy could also induce thyroid nodules and thyroid cancer [48]. The vast majority of radiation-induced solid tumors occur within the radiation field [35, 49]. In a study by Schneider and colleagues [43], 318 of 5,379 patients who had received RT for benign conditions of the head and neck developed thyroid cancer 3–42 years later. The most common type of cancer after thyroid radiation is papillary carcinoma, which, fortunately, has a high cure rate if detected early [50]. The course of the cancer in these patients appears to be the same as that of thyroid cancer found in other settings [43].
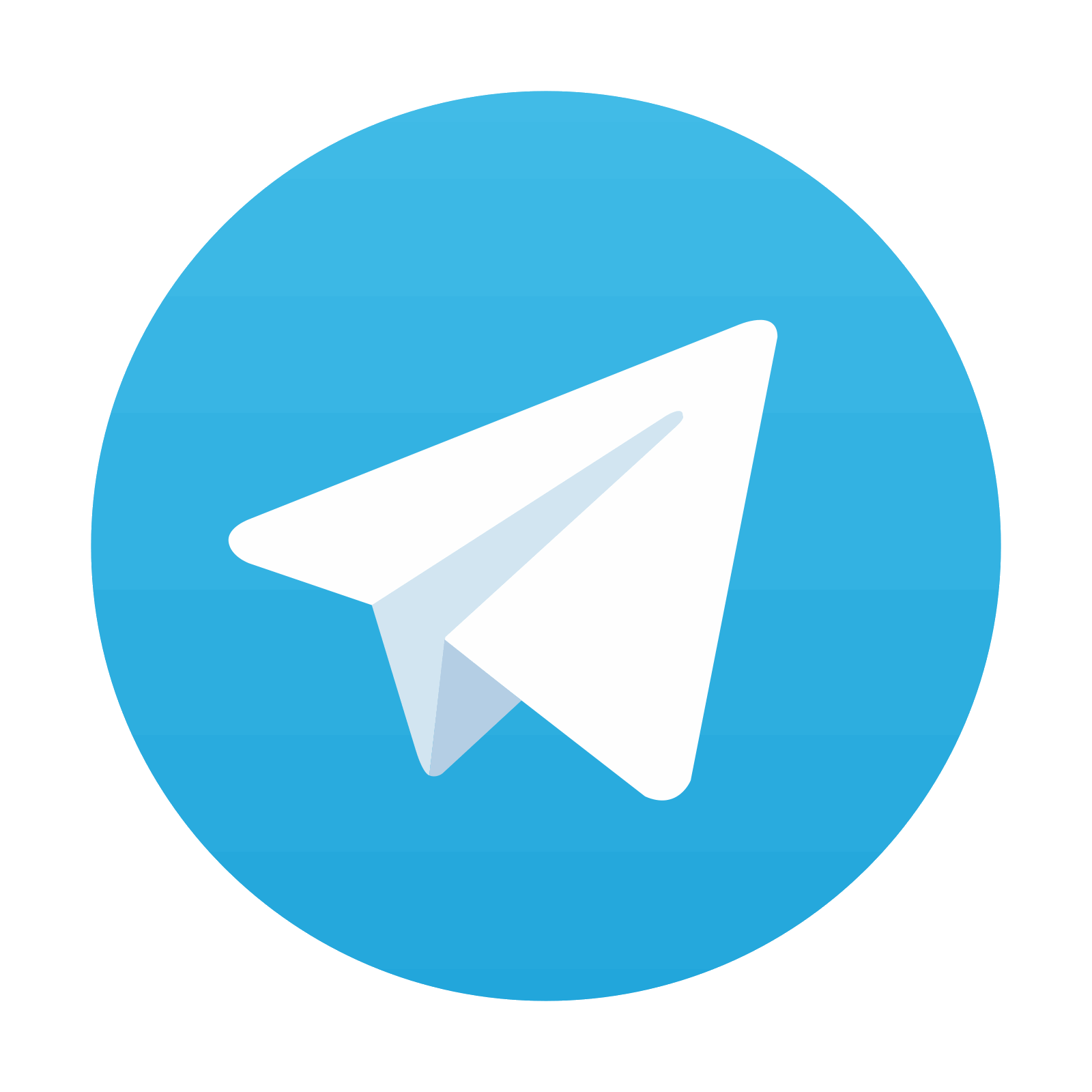
Stay updated, free articles. Join our Telegram channel
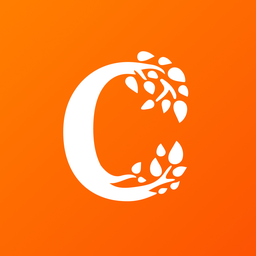
Full access? Get Clinical Tree
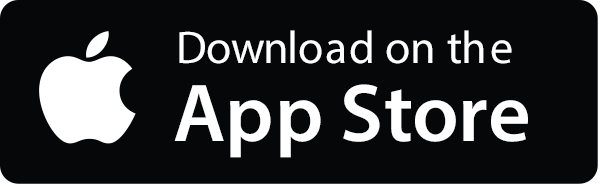
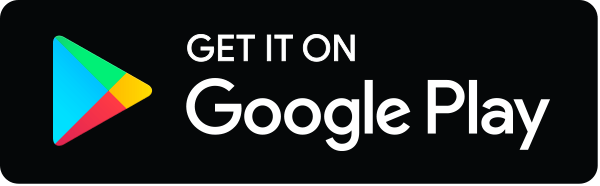