Fig. 16.1
Schematic of a growing long bone. The shaft of the bone is called the diaphysis and contains the medullary cavity, which is filled with bone marrow. The two expanded ends are the epiphyses. The epiphysis at each end extends from the articular cartilage to the epiphyseal growth plate. The metaphysis is the region between the epiphyseal plate and the diaphysis (From [64], Fig. 2.3, p. 8)
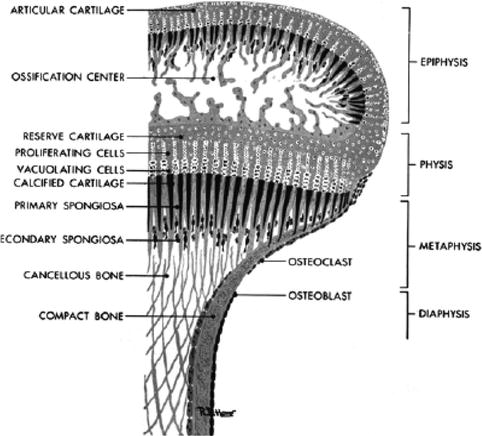
Fig. 16.2
A close-up of the region of the metaphysis and epiphysis. The proliferating cells (chondroblasts) are shown in the region of the physis (From [61])
Most skeletal muscles also develop before birth, although some muscle formation continues until the end of the first year of life. Cells from the myotome region of the dermomyotomes become elongated spindle-shaped cells called myoblasts. These embryonic muscle cells fuse to form multinucleated muscle cells called muscle fibers. The dermatome regions of the dermomyotomes give rise to the dermis of the skin [64]. No new muscle cells are created after that time; muscle tissue enlarges due to increases in the number of myofilaments within each fiber, which result in an increase in the diameter of the individual muscle cells.
16.2.1.2 Organ Damage Induced by Cytotoxic Therapy
The surgical removal of parts of muscles or bone can lead to severe functional deficits; however, direct damage to the developing musculoskeletal system from cytotoxic therapy is most often caused by irradiation. The cells most sensitive to irradiation appear to be the growth plate chondrocytes [28, 79, 90]. Low total radiation doses reduce cell mitosis, promote premature terminal differentiation and apoptosis, and completely disrupt the cytoarchitecture. Surviving chondroblast clones repopulate the physis (although not always completely) if the total dose is below 20 Gy. Above this level, little repopulation occurs. Bones show a high capacity for repair after damage and fracture. After the initial damage takes place, inflammatory cells infuse from blood, secreting transforming growth factor β (TGF-β) to activate mesenchymal stem cells in the bone marrow, which can differentiate into chondrocytes, adipocytes, and stromal cells. Initially, a soft tissue callus (procallus) forms around the ends of the fractured bone, and osteoblasts begin to deposit immature woven bone. Although osteoblasts are damaged only by high doses of RT, radiation quickly increases vascularity of the bone, particularly in the metaphysis. This response in turn increases the resorption of bone, thereby increasing its porosity and the demineralization of the immature metaphysis [67]. Mesenchymal cells in the procallus start to form hyaline cartilage, which will undergo endochondral ossification and develop into a bony callus. Bone remodeling will eventually change woven bone into mature lamellar bone.
Recent evidence shows that the effect of radiation is very specific and leads to a decrease in the messenger ribonucleic acid (mRNA) expression of parathyroid hormone-related peptide (PTHrP), which is an important stimulus for mitosis of chondrocytes. The mechanism that triggers the decrease of the mRNA appears to be an increase in cytosolic calcium. The introduction of ethyleneglycotetraacetic acid (EGTA), a calcium chelator, inhibits the rise of cytosolic calcium and prevents most of the radiation damage [72]. Several hormones have a clear influence on bone formation and remodeling, including growth hormone (GH), parathyroid hormone (PTH), calcitonin, thyroid hormones, androgens and estrogens, and cortisol. Vitamin D acts directly on osteoblasts to secrete interleukin 1 (IL-1), which stimulates osteoclasts to increase bone resorption. In animal experiments, pentoxifylline can also decrease the effect of radiation on growth plate chondrocytes by mediating a decrease in cytosolic calcium [73].
The major risk factors for producing musculoskeletal late effects secondary to irradiation are as follows: (1) age at the time of treatment, (2) quality of radiation (dose per fraction and total dose), (3) volume irradiated, (4) growth potential of the treated site, (5) individual genetic and familial factors, and (6) coexisting therapy – surgery or chemotherapy [24].
There are too many variables to determine the effect of each variable individually. The current literature would imply that there is no threshold dose and that any dose of radiotherapy will affect the growth plate. Doses greater than 20 Gy will usually result in complete arrest. Nevertheless, the response to radiation is not an all-or-nothing phenomena; the higher the total dose and the younger the age at treatment, the greater the ultimate deficit [94]. This dose effect is probably because the epiphyseal plate appears to close more quickly with increasing levels of dose (Fig. 16.3a–c).
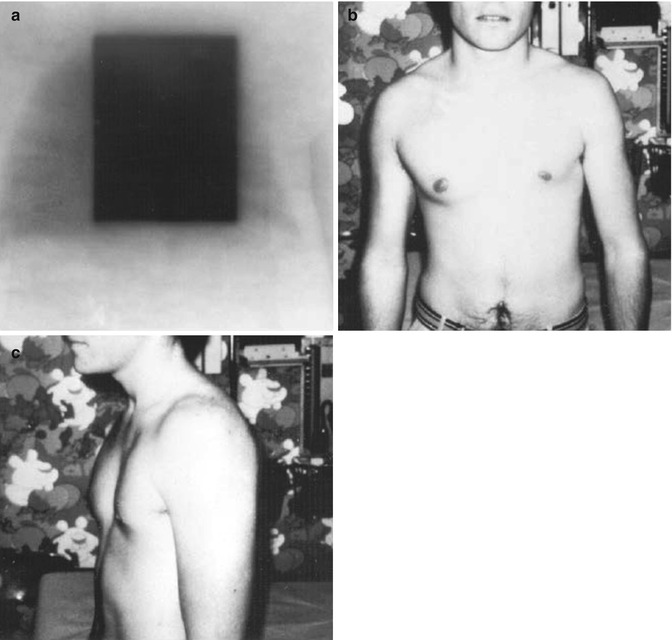
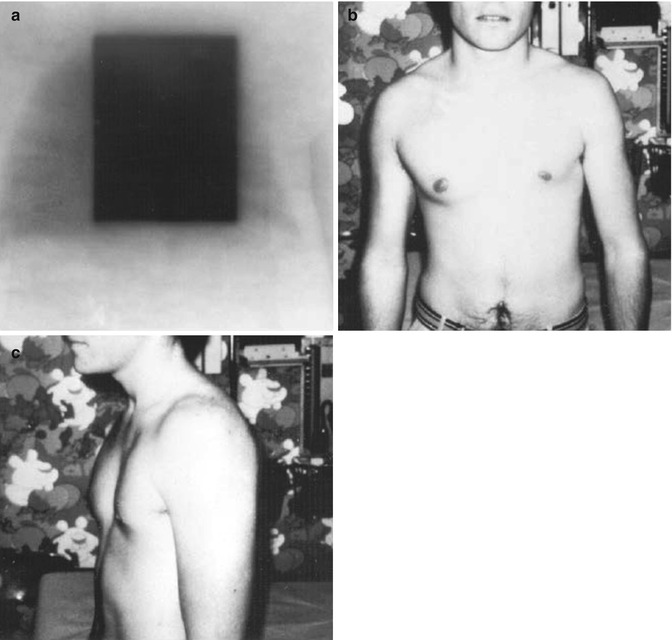
Fig. 16.3
(a–c) A 3-month-old boy who was treated in 1962 for a thoracic neuroblastoma using approximately 20 Gy in 10 fractions to the mediastinum and much of the left chest. (a) An AP-PA technique with 2-MEV photons was used. (b, c) The patient 20 years later. Mild hypoplasia of the left chest is present
Some authors report that permanent bone growth arrest can be observed after total doses as low as 12 Gy delivered to the growth plate [19]. Various degrees of deformities are also reported at lower dose levels (range, 20–35 Gy), without a definite dose threshold [60, 74]. Silber et al. created a model to predict growth deficits from spinal irradiation based on the vertebral bodies treated, the total dose at normal fractionation, and the age of the child [97]. From these data, significant deficits would not be expected below 20 Gy. Investigators at St. Jude Children’s Research Hospital (Memphis, TN) have attempted to use a random coefficient model for estimation of potential differences in volumetric bone growth in children with sarcoma treated with intensity-modulated radiation therapy (IMRT) and conformal radiation therapy (CRT) [45]. The model incorporated patient age, pretreatment bone volume, integral dose >35 Gy, and time since completion of radiation therapy and predicted that patients older than 10 years would maintain 98 % of normal growth, regardless of treatment method. Growth abnormalities are detected in almost all children treated with conventional fractionation to doses ranging from 40 to 55 Gy for extremity sarcomas [36, 74].
One of the goals of hyperfractionated radiotherapy (the use of small doses two or more times a day) is to decrease the extent of late effects. Studies are only now beginning to accumulate data, but Marcus and associates found that in the treatment of Ewing’s sarcoma, fractions of 1.2 Gy twice daily to 50.4–63.2 Gy produce fewer musculoskeletal late effects than would be expected from similar doses using fractions of 1.8–2.0 Gy once a day (Fig. 16.4) [11, 57].
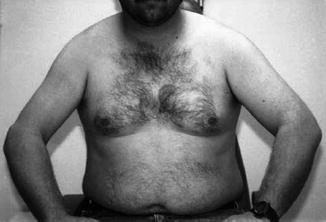
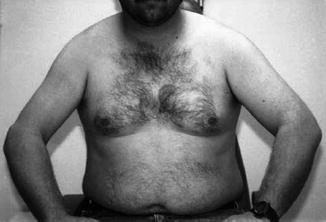
Fig. 16.4
This man was treated for Ewing’s sarcoma of the right humerus using 50.4 Gy in a fractionation scheme of 1.2 Gy twice daily when he was 14 years old. There was little noticeable difference in the muscle development in his upper arms until he started weight lifting. The left (untreated) arm responded, but the muscles in the radiation field did not increase as much in size or strength. In this picture, taken 11 years after treatment, the area of hypoplasia from the field of irradiation can be clearly seen
Other factors influence late sequelae. Orthovoltage irradiation, dose for dose, causes more growth deficits in the bone than megavoltage irradiation because of the increased bone absorption. Field size is also important: the larger the field of irradiation, the more significant the late effects [12].
Similar dose levels apply to muscle development as well. Doses <10 Gy cause virtually no detectable defect, and doses from 10 to 20 Gy produce some hypoplasia. Higher doses produce more significant problems.
Although high single doses of radiation can cause necrosis of muscle cells, in clinical practice, such an event is extremely rare. More commonly, even with fractionated treatment, radiation damages the small vessels in muscles, preventing the full development of the muscle due to relative ischemia. Higher doses can give rise to atypical fibroblasts, which lay down excessive fibrin in the tissues, causing fibrosis [114].
There are sparse data on the pathophysiology of damage to growing muscle and bone by cytotoxic drugs, although in animal models the use of chemotherapy has caused a reduction in the shear strength of the physis [7, 104].
Chemotherapeutics have been shown to retard the proliferation of growth plate chondrocytes in both in vitro and in vivo experiments [85]. Chemotherapy retards growth during the course of treatment, but after the end of active therapy, most reports indicate that patients return to their normal growth rate or even exhibit catch-up growth [78]. Histologic examination of human growth plates after neoadjuvant chemotherapy and surgical excision has shown maintenance of the overall epiphyseal architecture, with growth arrest and then resumption of growth [7]. Most evidence would indicate that the deficit is temporary or primarily caused by endocrine dysfunction [6, 14].
Methotrexate is a chemotherapeutic agent known to be associated with growth stunting, osteoporosis, and fracture [63, 66, 70]. The underlying pathophysiology for osteopathy in pediatric cancer patients receiving methotrexate chemotherapy remains unclear; however, animal experiments support the evidence that methotrexate interrupts bone resorption, inhibits bone turnover, and reduces recruitment of osteoblastic cells from proliferative precursor [29].
High or prolonged courses of steroids can cause the development of avascular necrosis of the femoral head. The exact mechanism is poorly understood, but there is evidence in animals to support a temporary decrease in blood flow through bones during treatment with steroids. Because the vasculature of the femoral and humeral heads is fragile, these structures are the most easily damaged by the decrease in blood flow, resulting in necrosis [25]. A class of drugs called bisphosphonates is used to treat patients with osteolytic metastasis, reducing skeletal complications by disruption of osteoclast-mediated bone resorption leading to decreased bone turnover. Some patients exposed to bisphosphonate therapy develop osteonecrosis [58, 113]. While the exact mechanism of osteonecrosis is still not known, the pathogenesis of localized vascular insufficiency may be attributable to release of cytokines; interactions with growth hormone and insulin-like growth factor are known to play a role in regulation of blood circulation in bones, possibly from direct inhibition of endothelial cells. Metaphyseal sclerotic banding is another documented effect of periodic bisphosphonate treatment in growing children [110].
There is emergence of evidence about risk of osteonecrosis in patients treated with antiangiogenic drugs (bevacizumab), increasingly used in pediatric patients with newly diagnosed and recurrent malignancies. Rates of mandibular osteonecrosis have been increasingly observed in a combination of bevacizumab and bisphosphonates [13]. Recent evidence suggests that children treated with bevacizumab can be at risk for the development of lytic lesions and frank osteonecrosis in long bones [30, 98].
Retinoid acid derivative 13-cis-retinoic acid is used to treat minimal residual disease in patients with high-risk neuroblastoma. This agent is known to be associated with premature epiphyseal closure, and children were noticed to develop advanced bone age [40].
A more serious complication is rhabdomyolysis, a rare complication of cytarabine and other drugs, including cyclophosphamide, 5-azacytidine, interferon-α, and interleukin-2 [103]. It is thought that the ability of cytarabine to trigger the release of cytochrome-c from the mitochondria could lead to uncoupling of the oxidative phosphorylation with subsequent depletion of adenosine triphosphate (ATP) reserves at the skeletal muscle, resulting in rhabdomyolysis. Whatever the cause, the syndrome is complicated by acute renal failure often requiring hemodialysis.
16.2.2 Clinical Manifestations
Surgery, radiation therapy, and chemotherapy can all produce long-term sequelae in the developing musculoskeletal system; the effects of surgery and radiation have been more thoroughly studied than those of chemotherapy.
Most of the following text will focus on the late effects of irradiation; however, it is important to remember that the surgical removal of a portion of the musculoskeletal system can result in the same physical and psychological late effects as damage to the same tissue from radiation therapy or chemotherapy. The loss of a muscle group in the proximal lower extremity will cause weakness and gait disturbances; the amputation of an entire extremity will require the abrupt need for extensive rehabilitation. Limb length discrepancies often result from the unilateral surgical removal of one or more growth plates. The fact that the deficit is planned does not imply that the late effects should be discounted.
16.2.2.1 Bone
Some of the adverse effects of cancer therapy on growing bone are (1) bone density changes, (2) physical injuries, (3) pathologic fractures, and (4) avascular necrosis/osteonecrosis.
Bone Density Changes
Radiation therapy can produce osteopenia in children. More information is needed to elucidate all the parameters involved, but the risk is probably between 8 % and 23 % at doses above 20 Gy, primarily seen in patients with cranial irradiation and could be attributed to growth hormone deficit. However, bone mineral loss up to 7–8 % can be detectable after 30 weeks following 20–25 Gy irradiation in rat femurs. The relationship between osteopenia and pathologic fracture remains unclear [43].
Physeal Injury
The physical effects of radiation therapy are secondary to damage to the chondroblasts. This damage results in a slowing of, or arrested, physical growth, producing abnormal development of the involved bone, giving rise to (1) spinal abnormalities, (2) leg or arm length discrepancies, (3) angular deformities at joints, (4) slipped capitofemoral epiphyses, and (5) osteocartilaginous exostoses.
Spinal Abnormalities
Spinal abnormalities most commonly include decreased stature or scoliosis, although lordosis or kyphosis can also occur [12, 67]. Except for decreased growth, severe spinal complications are less common after megavoltage RT, compared with the orthovoltage era, if the entire vertebral body is within the treatment field [83]. Although curvature can occur, it is usually a result of tethering that occurs with decreased muscle development as a result of irradiating just one side of the abdomen, as is often done in Wilms’ tumor.
On standard radiographs, vertebral bodies show subcortical lucent zones within 9–12 months after completing RT. The subcortical lucent zones progress over the next 1–2 years to form growth arrest lines, which parallel the epiphysis of the vertebral body [91]. Following the development of these arrest lines, little or no further growth occurs. The higher the dose, the quicker the growth arrest occurs. Other changes may also be seen on radiographic examination. During the first few months after irradiation, vertebral bodies may have a more bulbous contour [49]. Scalloping of the physical cartilage plates may also be observed, and not infrequently, the final appearance is that of rounded vertebral bodies with central beaking [91].
Clinically, the deleterious effect of radiation on growing bone has been known for a long time. Neuhauser and associates reported on 24 children who received orthovoltage irradiation to the spine during their treatment for Wilms’ tumor or neuroblastoma [67]. Doses below 10 Gy (using orthovoltage irradiation) caused no detectable vertebral abnormality regardless of the age at treatment. Higher doses caused severe late effects in the spine. Mayfield and coworkers reported on 28 children with neuroblastoma who received irradiation to their spine [60]. Scoliosis was the most common sequela and occurred most severely at doses above 30 Gy with orthovoltage irradiation. Megavoltage irradiation appears to cause fewer severe orthopedic complications. Rate et al. reported on a series of 31 Wilms’ tumor patients irradiated between 1970 and 1984. Three out of ten patients treated with orthovoltage irradiation developed late orthopedic abnormalities requiring intervention [82]. None of the 21 patients receiving megavoltage treatment developed such complications, even at doses of 4,000 cGy. Scoliosis was related to a higher median dose (2,890 cGy) and a larger field size (150 cm2); this sequela did not result for patients receiving smaller doses to a smaller field size (2,580 cGy–120 cm2). Paulino et al. report a lower incidence of scoliosis with doses <2,400 cGy than with higher doses [77]. Both orthovoltage and megavoltage irradiation were used. Probert and Parker were the first investigators to attempt to evaluate bone growth alterations secondary to megavoltage irradiation. They noted deficits sitting height for patients who received radiation to the spine for medulloblastoma, leukemia, and Hodgkin’s disease [79]. Doses greater than 35 Gy were found to produce a significantly greater deficit in sitting height, compared with doses less than 25 Gy.
Silber and coworkers, using data from 36 children whose spine or pelvis was irradiated, have developed a mathematical model for predicting stature loss [97]. The model is based on the radiation dose in Gy, the location of the therapy (including whether or not the capitofemoral epiphysis was treated), gender, and the ideal adult height. Figure 16.5 shows an example of the model, based on four different irradiation doses. The model agrees closely with a report by Hogeboom et al. who calculated the height deficit after flank RT for Wilms’ tumor patients at different ages and doses of radiation therapy [41]. In both reports, even a dose of 10 Gy at age 2 produced a height deficit of 2.3–2.4 cm by age 18 years. For infants less than 12 months of age receiving 10 Gy or more, the estimated height deficit was 7.0 cm. Although chemotherapy did not appear to increase the effect of radiation therapy in Hogeboom’s study, there was a deficit associated with doxorubicin that – although it could be explained, at least partially, by other factors – does not completely rule out a permanent doxorubicin effect on bone growth.
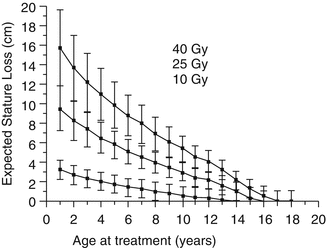
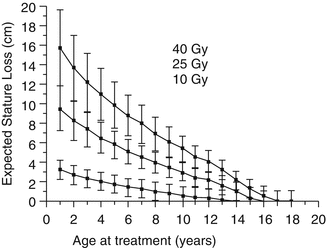
Fig. 16.5
An example of the model for expected stature loss after radiation therapy to the spine during childhood, as proposed by Silber and coworkers. A hypothetical male patient was treated from T10–11 to L4–5, and his ideal adult stature was 176.8 cm. Each point corresponds to the age when irradiated, the dose in Gray, and stature loss, plus or minus one standard deviation (From [97], p. 309)
Limb Length Discrepancy/Angular Deformity
Irradiation of the extremities or hip usually produces more long-term symptomatic sequelae than irradiation of the spine, particularly when an epiphysis is treated. Radiographic changes first reveal metaphyseal irregularities and epiphyseal widening. Later changes include sclerosis around the physis and eventually sclerosis and closure of the epiphyseal plate. Such premature closure can cause a discrepancy in ultimate length between the irradiated and unirradiated extremity. The degree of growth disturbance in long bones is dose and volume dependent; moreover, patient age plays a very important role [35]. In addition, if the entire physis is not included in the radiation port, then juxta-articular angular deformities can result [84]. It is possible to predict the effects of radiation on growth based on age and growth pattern. Altogether, about 65 % of leg growth occurs at the knee, 37 % from the distal femoral physis, and 28 % from the proximal tibial physis. Only 15 % occurs at the proximal femoral growth plate and 20 % from the distal tibial plate [5, 36]. This estimate may not be completely accurate because it assumes that no growth occurs after irradiation of the growth plate, but in reality, growth continues for at least a short period of time. Additionally, untreated physis in the same extremity can partially compensate for the affected one. Asymmetric dose delivery to the metaphysis can cause valgus and varus deformities [31].
Surgery may also have a significant effect on limb function. Limb preservation with expandable prosthesis has become a widely adopted method in the local management of bone malignancies in children [2, 10]. Repeat procedures for prosthesis, either lengthening or replacement, is often required [26], which potentially adversely affects the physical and emotional well-being of children [39, 102]. Rotationplasty is another durable reconstructive procedure which is associated with significant limb length discrepancy requiring prosthetics. Despite its complexity, children receiving this treatment appear to have reasonable physical and emotional resilience [32, 34]. Amputation is the most radical surgical procedure associated with the loss of a significant portion of the limb and can only be compensated with prosthetics. Despite significant differences between limb sparing, rotationplasty, and amputation, data suggests that survivors have fairly comparable physical and emotional outcomes with either approach [44].
Craniofacial Deformities
Microcephaly, micrognathia, and other growth abnormalities are reported in children with retinoblastomas and rhabdomyosarcomas receiving external beam radiation to the craniofacial region [24, 62, 81].
Surgical management of tumors in the head and neck of young children may be associated with even more substantial cosmetic and functional disturbances. Given the anatomical challenges of these tumors, there is often concern about leaving microscopic or gross disease behind; thus, adjuvant radiotherapy is given [18]. This combined approach can result in more significant dysfunctions due to worse fibrosis developing over time. Reconstructive surgeries can correct these problems to an extent, but long-term outcomes are still far from satisfactory [16]. Anthropometric and descriptive methods are commonly used for documenting the extent of deformities, but they are hard to quantify. Severe hypoplasia or asymmetry of tissues causing cosmetic and functional problems may require reconstructive surgery.
Slipped Capitofemoral Epiphysis
Radiation therapy has been shown to increase the risk for slipped capital femoral epiphysis due to epiphyseal plate injury [9, 108, 112]. Patients with slipped capitofemoral epiphyses present with pain in the hip or knee (referred from the hip). The pain presents through either acute or chronic onset, since slippage of the capitofemoral epiphysis often proceeds slowly. Wolf and associates first reported cases of slipped capitofemoral epiphysis as a result of childhood irradiation [112]. These occurred 1–6 years after therapy with doses of 28.5–54 Gy and usually after the onset of puberty when there is a change in the angle of the femoral shaft in relation to the femoral neck and head (a change that increases the susceptibility to stress from excess weight and other factors). Children developing a slipped epiphysis after irradiation were generally 2–3 years younger than the average patient with idiopathic-slipped capitofemoral epiphysis, had twice the risk of bilateral involvement (20–50 %) if both proximal femurs were exposed to radiation, and, furthermore, did not usually fit the generally obese body habitus of the average patient presenting with the idiopathic variety. Paulino et al. reported on four infants (<6 months old) irradiated to the hip to doses of 20 Gy or higher for a neuroblastoma [75]. Two patients developed slipped capitofemoral epiphysis, at 25.5 Gy and 36 Gy. The other two patients received doses of 20 Gy and did not develop the problem. Slippage of the physis is thought to occur as a result of excess stress, either from obesity or from a weakening of the bone and physis secondary to radiation therapy. The threshold dose is thought to be around 25 Gy.
The use of chemotherapy has caused a reduction in the shear strength of the physis in an animal model [104]. Theoretically, chemotherapy would also appear to predispose patients to developing slipped capital femoral epiphysis, but this has not been studied in humans.
Exostosis
Osteocartilaginous exostoses are benign outgrowths of the physis. They have been reported to occur in up to 18 % of children treated with radiation therapy [3], although the incidence in the megavoltage era is much lower. The etiology is unknown, but is thought to be due to an injury to the periphery of the growth plate. The lesion is a combination of a radiolucent cartilaginous cap with areas of ossification and calcification. It has a typical cauliflower appearance and the base may be narrow or broad. On physical examination, a hard mass is palpable adjacent to a joint [94]. Lesions may continue to grow slowly throughout childhood, although by the third decade growth should cease.
There is a small incidence of malignant degeneration that presents with pain and occurs after skeletal maturation.
Pathologic Fractures
Irradiation of the metaphyseal portion of the bone may temporarily cause increased porosity and demineralization; radiographic examination will show cortical thinning and irregularities during this time. The irradiated segment of bone therefore acts as a stress concentrator and predisposes the bone to fracture [38]. This effect is particularly true in patients in whom the irradiated bone has been weakened by tumor involvement, such as in Ewing’s sarcoma of the humerus and femur. This effect also occurs if the cortex of the bone has been biopsied, particularly in the area of the femoral neck [107]. Pathologic fractures usually occur within 3 years after treatment [38]. The most common site is the upper third of the femur, and since fractures can be caused by tumor recurrence, a full reevaluation of the patient should be performed to ensure that it is not related to relapse [107].
Avascular Necrosis/Osteoradionecrosis
Avascular necrosis (AVN) of the femoral head has been reported as a complication of hip irradiation in children who have received doses exceeding 30 Gy [53]. Older age (usually >10 years old) appears to increase the risk, indicating that the rapidly growing and maturing bones of adolescents are more susceptible to the development of AVN [59]. High cumulative corticosteroid doses administered during the therapy strongly predispose patients to AVN, and dexamethasone seems to be more toxic than prednisone. AVN may be asymptomatic or result in joint swelling, pain, limited range of motion, and even joint damage and articular collapse. MRI is the best technique to detect early stages of AVN. The typical radiographic picture is shown in Fig. 16.6a–d.
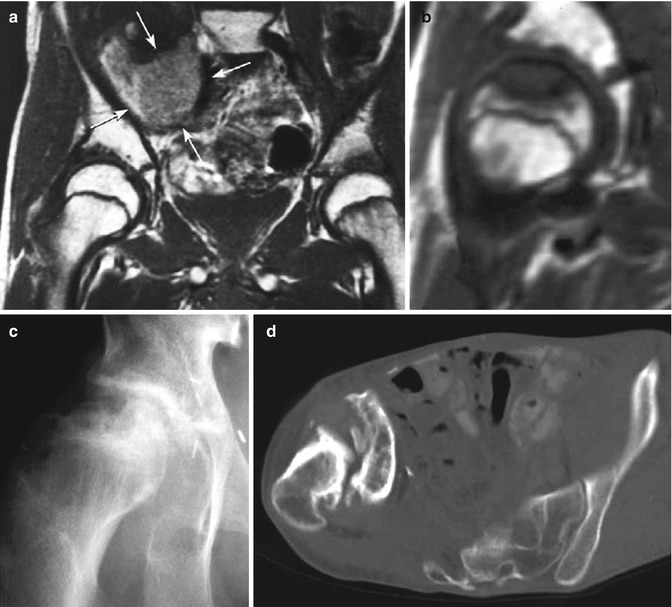
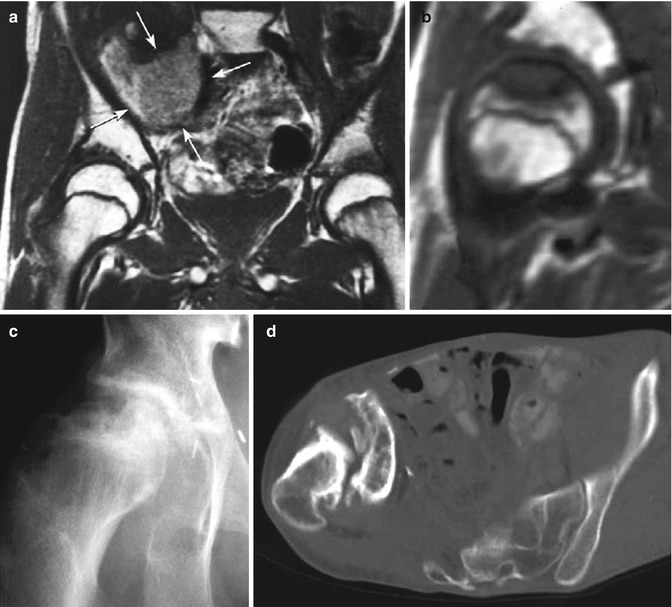
Fig. 16.6
(a–d) A 14-year-old boy treated with preoperative irradiation (61 Gy) and chemotherapy for a synovial cell sarcoma of the right iliopsoas muscle. (a) Tumor (arrows) and normal femoral heads. (b) An MR scan 6 months later showing a close-up of the right femoral head. The scalloped, non-enhancing lesion in the femoral head is typical for an osteonecrosis. (c) A plain radiograph 1 year later, showing healing of the femoral head (b–d, see next page). (d) The early healing was not permanent, however, and a computed tomography scan 13 years after treatment demonstrates collapse of the femoral head, chronic dislocation of the femoral head, and extensive degenerative changes. Severe scoliosis resulted as well
16.2.2.2 Muscle
The most common late effect of developing muscle tissues secondary to irradiation is diminished development of the muscle tissues (hypoplasia). The muscles treated are smaller and functionally not as strong as the patient’s unirradiated muscle tissues. Still, the differences in strength are not pronounced, and, for the majority of patients, it is more of a cosmetic problem than a functional one. Nevertheless, it is a common problem. Macklis et al. reported that 13 of 14 long-term survivors of Wilms’ tumor treated with low-dose pulmonary irradiation had musculoskeletal hypoplasia [55]. Long-term survivors treated with head-and-neck radiation therapy are reported to have a 77–100 % incidence of mild to severe radiation damage of soft tissues and bones [76, 80]. Radiotherapy for soft tissue sarcoma of the limb conventionally involves irradiation of the entire transverse cross section of the affected anatomical compartment [8]. Studies have shown that the functional outcome after radiotherapy to the limb is related to the radiotherapy volume and the size of the unirradiated corridor [4]. The total dose and dose per fraction of radiotherapy used are also a significant factor in determining range of movement, muscle power, and limb function [50]. Posttreatment function is paramount in the management of soft tissue sarcoma.
With higher doses, these tissues can develop marked fibrosis, which can produce stiffness, a decrease in range of motion of a joint, and even pain [88]. In mild cases, stiffness or pain occurs primarily in the early morning and is improved by use of the involved muscles. Occasionally, the patient may have pain that is more persistent, lasts all day, and, even with use, does not improve.
A specific type of fibrosis is trismus, which is an inability to fully open the mouth. When the masticator muscles and the temporomandibular joint are included in the irradiated field, musculoskeletal fibrosis can cause trismus and mandibular dysfunction. It has been reported in about 5–10 % of children treated for head-and-neck malignancies [71, 105]. Since doses are lower in RMS of the head and neck, trismus is less common in RMS than in nasopharyngeal carcinoma [76].
16.2.3 Impact of Aging
In general, growth deficits of the musculoskeletal system from irradiation are amplified with increasing years after treatment. Obviously, when all epiphyses are closed, further bone growth deficits do not occur. However, other late effects of bone will continue to occur, and muscular hypoplasia (and fibrosis) may become more significant.
16.2.4 Detection/Screening
A thorough history and physical examination is critical in the evaluation of musculoskeletal treatment sequelae. It is necessary to know exactly when a deformity becomes symptomatic, the symptom complex, and the details of the previous cytotoxic treatment related to the deformity. Attention should be given to the types of surgical procedures, location of the radiation fields, radiation doses used, and details (including doses) of the chemotherapy regimen.
The physical examiner should look for skin changes from the cytotoxic treatment as well as any obvious deformity. The musculoskeletal assessment should include serial measures of weight, height, and sitting height (crown to rump). These measurements should be plotted on growth velocity charts to assess whether growth rate is within normal limits. Most normal children will grow a minimum of 5 cm per year between age 3 years and puberty. A patient in that age group who grows <5 cm per year, or whose serial heights on growth charts begin to fall into the lower percentiles, may be experiencing growth failure [65].
Any joints involved in the radiation fields, or affected by surgery, should be put through passive and active ranges of motion, and comparisons should be made with the contralateral side. Joint measurements should be taken if indicated by abnormalities in “performance” range of motion – for example, if the active motion of a particular joint is less than the passive range of motion. In the event that there are performance range-of-motion abnormalities, further assessments are indicated. Other joint problems that can occur include pain, crepitation, swelling, loss of mobility, and weakness [56].
Observation of gait and posture must be included in the musculoskeletal assessment. As indicated by treatment received, the muscles to be examined can be assessed by functional groups, and comparisons can be made bilaterally for symmetry, tone, size, and strength. Any areas of deformity, swelling, atrophy, or weakness should be noted [65].
The patient should be assessed for level of functioning and participation in normal daily activities, such as school and after-school activities. Normal growth and development parameters should be incorporated into this assessment, as developmental stages may influence the patient’s participation in some activities. Another influence on the level of functioning or decreased participation in activities could be the lack of adjustment to body image changes.
There is no current method of completely preventing the development of late musculoskeletal effects from surgery or radiotherapy. The patient who has experienced an amputation or a significant growth deficit due to radiotherapy may or may not have incorporated this long-term effect into a new, positive body image. Many other factors contribute to general growth and development, such as nutritional deficits, other tissue damage, and hormonal influences. The examiner should not rush to attribute the entire problem to the cytotoxic treatment [65]. If the right assessment is made, intervention can sometimes prevent an asymptomatic or mildly symptomatic problem from becoming more clinically significant. At the very least, the appropriate intervention may be able to assist the patient in adapting to body image changes.
16.2.4.1 Spinal Sequelae
The evaluation of spinal sequelae should include the region of curvature, the magnitude of the curve, the deviation from vertical, the degree of shoulder asymmetry, the position of any rib humps or rib flare, and the type and degree of any gait abnormality. Usually, the best way to examine the back is with the patient bending over with the arms touching the toes and the knees straight. At each visit, measurements should be taken of the standing and sitting heights. The spinal shortening that occurs as a direct effect of irradiation is not correctable, but, except for an ultimate decrease in height, does not usually cause major problems, unless spinal curvature develops. Anteroposterior and lateral films of the entire spine should be used to screen for this. It is also important to be able to inform the patients of the height deficit to be expected. Figure 16.5 shows a model of expected stature loss by age at treatment for three dose levels for a hypothetical male patient receiving radiation from T10–T11 to L4–5 [97].
If the spine has been irradiated, standard radiographic images should be taken every 1–2 years until skeletal maturity to detect early scoliosis or kyphoscoliosis (Fig. 16.7). After that, films should still be taken every 1–2 years if some curvature is already present. It is rare to develop curvature after skeletal maturity if none was present before.
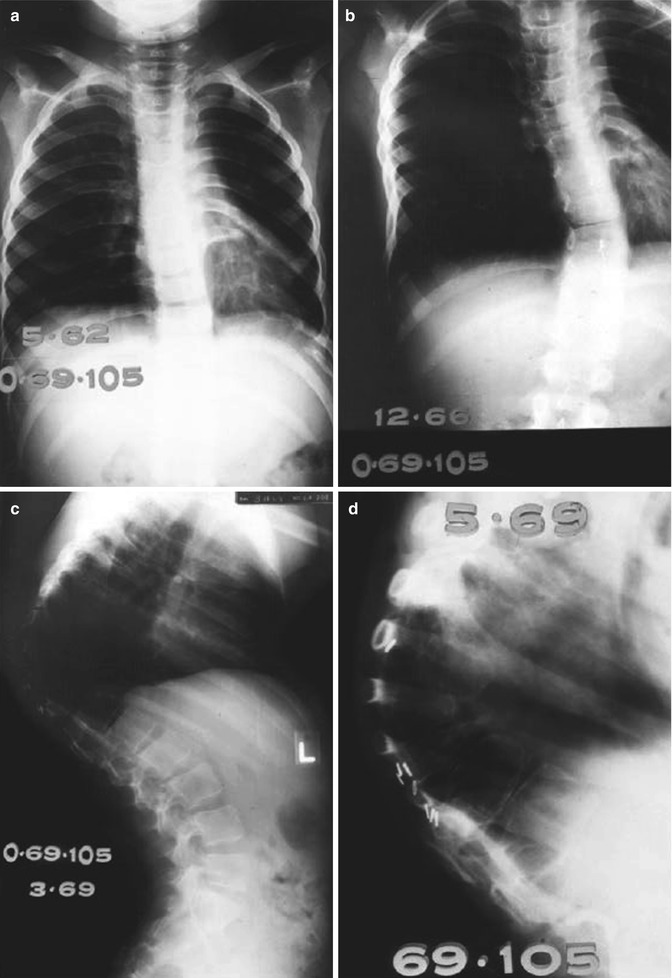
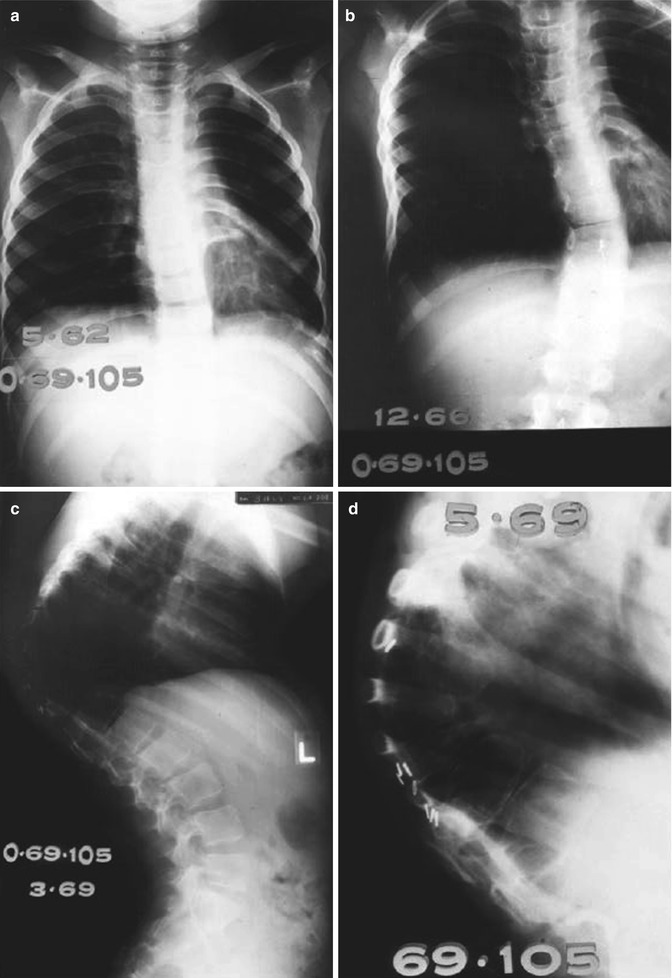
Fig. 16.7
(a–d) A 2-year-old girl treated with orthovoltage irradiation to the abdomen and spine (dose unknown) for a neuroblastoma in 1960. (a) Two years after treatment, mild scoliosis developed. (b–c) Progression of kyphoscoliosis occurred over the next 7 years. (d) A close-up of the spine 9 years after therapy shows osteoporosis of vertebral bodies and wedge-shaped compression fractures
The most common method for measuring spinal curvature is the Cobb technique. The two end vertebrae of the curvature, the ones most tilted from the horizontal on the upright film, are selected. A line is drawn along the upper end plate of the upper end vertebra and along the lower end plate of the lower end vertebra. The angle of intersection of the perpendiculars from these lines is the angle of the curvature (see Fig. 16.8). It is extremely important to perform these measurements carefully. Since progression of any defect may be more important than the occurrence of the defect, the amount of curvature, both sites should be measured.
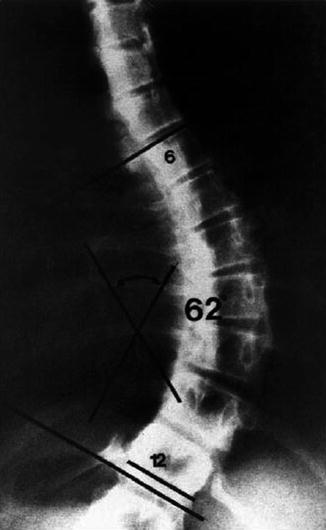
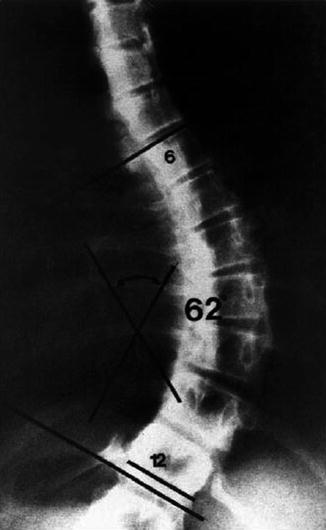
Fig. 16.8
The Cobb technique for measuring the angle of spinal curvature. See text for a description (From Winter [111])
16.2.4.2 Limb Length Discrepancy
Limb length discrepancies are usually more significant. Differences in length between upper extremities are not often a problem, but leg length discrepancies can cause significant functional deficits. It is therefore important to be able to predict the ultimate outcome when radiotherapy is chosen. To do so, knowledge of the future growth of all epiphyses is necessary. In the lower extremity, 65 % of future growth comes from the knee, 37 % from the distal femoral physis, and 28 % from the proximal tibial physis. Only 15 % occurs at the proximal femoral plate and 20 % from the distal tibial plate [65]. Table 16.1 provides rough estimates, by age of the patient, of the growth remaining for the four major lower extremity epiphyses. Information such as this can be used to calculate the probable discrepancy that may develop assuming that there is no growth of the irradiated physis after treatment. This is not completely accurate, since some growth may occur for a short time, and other untreated growth plates in the same extremity may partially compensate for the closed physis.
Table 16.1
Average growth (in cm) remaining for each lower extremity epiphysis by age and sex
Epiphysis | Boys (age in years) | Girls (age in years) | ||||||||
---|---|---|---|---|---|---|---|---|---|---|
8 | 10 | 12 | 14 | 16 | 8 | 10 | 12 | 14 | 16
![]() Stay updated, free articles. Join our Telegram channel![]() Full access? Get Clinical Tree![]() ![]() ![]() |