INTRODUCTION
SUMMARY
Regenerative medicine is a complex and rapidly advancing field that holds tremendous promise in treating, and even curing, many diseases. The understanding and control of tissue repair is one of the most urgent challenges in medicine today. Regenerative medicine seeks to either recruit the patient’s reparative cells or to replace the malfunctioning tissue altogether to restore the deficient organ to adequate function. The common link among all types of regenerative therapies is the stem cell, which gives all tissues the capacity to regenerate. The mechanisms underlying the ability of a progenitor cell to differentiate have been challenging to elucidate, with recent experimentation focused on editing the genome itself. It has been even more difficult to determine how a differentiated cell can be instructed to revert to an immature state and undergo a re-specification to another differentiated cellular phenotype or an asymmetrical division to generate more immature cells. Our ability to modify genomes, harness stem cells, and transplant autologous or allogeneic tissues has transformed biomedical inquiry and offers hope to patients with diseases spanning all organ systems, including cardiac, lung, central nervous system, and liver and pancreatic diseases.
Acronyms and Abbreviations:
ALS, amyotrophic lateral sclerosis; AMI, acute myocardial infarction; ATI or ATII, alveolar epithelial cells type I or II; BASCs, bronchiolar alveolar stem cells; BDNF, bone-derived neurotrophic factor; BM-derived, marrow-derived; CAR, chimeric antigen receptor; CDCs, cardiac-derived stem cells; COPD, chronic obstructive pulmonary disease; CRISPRs, clustered regularly interspaced short palindromic repeats; dmPGE2,16,16-dimethyl-prostaglandin E2; DPSCs, dental pulp stem cells; DSB, double-strand break; EC, embryonic carcinoma; ESCs, embryonic stem cells; EPCs, epithelial progenitor cells; FAH, fumarylacetoacetate hydrolase; GVHD, graft-versus-host disease; HCT, hematopoietic cell transplantation; hESC, human embryonic stem cell; HR, homologous recombination; IDLV, integrase-deficient lentiviral; iPSCs, induced pluripotent stem cells; MN, meganuclease; MNCs, mononuclear cells; MSCs, mesenchymal stromal/stem cells; NHEJ, nonhomologous end-joining; NSC, neural stem cell; OPCs, oligodendrocyte progenitor cells; OT, off target; PD, Parkinson disease; SCID-X1, X-linked severe combined immunodeficiency; SCNT, somatic cell nuclear transfer; TALEN, transcription activator-like effector nuclease; TCR, T-cell receptor; TGF-β1, transforming growth factor-β1; UBCs, umbilical cord blood cells; VEGF, vascular endothelial growth factor; ZFN, zinc finger nuclease.
Regenerative medicine is a concept that evolved from knowledge in genome regulation and modification, from understanding of embryonic development and “stemness” of cells, and from 50 years of experience in human transplant biology. Therefore, a narrow view of any of these disciplines is not sufficient for illuminating the mechanisms of action underlying the already accomplished successes and for guiding the potential of novel basic biology discoveries into clinically meaningful regenerative medicine (Fig. 30–1).
Figure 30–1.
The three-body problem of regenerative medicine. The three factors—cell, genome, and patient—influence each other in complex and sometimes unexpected ways. These three separate scientific foci of regenerative medicine must be developed in the context of one another to have meaningful impact.
Accordingly, this chapter spans major organ systems (marrow, liver, pancreas, brain, and spinal cord) to demonstrate their connectivity and shared biologic responses deployed at the times of acute and chronic injury. Furthermore, the goals of regenerative therapies are different than those of commonly used drugs. Medications are typically aimed at amelioration of symptoms, while regenerative medicine seeks to either recruit the patient’s reparative cells or to replace the malfunctioning tissue altogether to restore the deficient organ to adequate function.
Regenerative medicine harnesses the body’s own repair mechanisms to replace, restore, or regenerate damaged or malfunctioning cells and tissues in conditions as diverse as diabetes, heart disease, spinal cord injury, and types of blindness. Some regenerative medicine therapies are already in use, for example using unrelated hematopoietic cell transplant to regenerate a patient’s immune system after their marrow has been destroyed by chemotherapy or radiation. There are some therapies that are in the early stages of clinical trials, for example using a patient’s cells seeded onto a biomesh scaffolding to grow a new trachea, ear, or nose. Some therapies are on the cusp of progressing into clinical trials, such as differentiating human embryonic stem cells into beta cells that could produce insulin in diabetic patients. And some therapies, such as growing new lungs from patient cells and repairing a spinal cord injury with a cellular bridge, remain tantalizingly out of reach.
The zygote has the ability to give rise to a complete organism. Any cellular genome in the organism has the ability to code for any protein in the body. Although we know this, the mechanisms underlying the ability of a progenitor cell to differentiate have been challenging to elucidate. For the earliest critical steps in this long and complex process, we must look at developmental biology. Nuclear transfers in amphibians done by Briggs, King, and Gurdon1,2,3 established that bidirectionality of cellular fate determination is possible. It was, established by McGrath and Solter that this process is driven by a multitude of factors of such temporal and spatial complexity that it would make reprogramming of mammalian cells by nuclear transfer impossible.4,5 Recent experimentation has focused on editing the genome itself, finding success in both mouse and human DNA models. Much work remains to bring this technology into human therapies, but in the foreseeable future, cells and organisms will no longer be seen as being given sealed orders at birth, but rather the instructions contained in their developmental program can be thought of as “software” that can be rewritten and used to reprogram the genomic “hardware” of a cell.
It has been even more difficult to imagine and later define the possibility that a differentiated cell could be instructed to revert to an immature state and undergo a respecification to another differentiated cellular phenotype or an asymmetrical division to generate more immature cells. Yamanaka’s experimental proof reduced this perceived complexity to a four-factor recipe sufficient to restore skin fibroblasts to induced pluripotent stem cells (iPSCs).6,7,8
This chapter gives a broad overview of the state of regenerative medicine as it stands today, a complex and rapidly advancing field that holds tremendous promise in treating, and even curing, many of the disorders that cause pain and suffering.
MULTIPOTENTIAL CELLS
Four to 5 days after fertilization, an egg becomes a blastocyst, a ball of approximately 100 to 150 cells. A small group of inner cells within the blastocyst are pluripotent and have the potential to replicate indefinitely and to become any of the differentiated types of tissue in the body. These pluripotent cells are called embryonic stem cells (ESCs). ESCs have the dual ability to self-renew (copy themselves) and differentiate (produce more specialized types of cells of the body).
ESCs were first isolated from mice when embryonic carcinoma (EC) cells9 were shown to proliferate indefinitely like stem cells and were used to generate a chimeric mouse. Further development of the culture conditions for EC cells that used feeder (supporting) cells, along with discovery of cell-surface antigens, like SSEA-1 and F-9 antigen on EC cells, led to the isolation of the first ESCs from a mouse embryo in 1981.10 In 1995, Thomson isolated ESC lines from a nonhuman primate,11 followed by the first successful isolation of human embryonic stem cell (hESC) lines in 1998.12 The use of human ESCs in research, however, has been severely limited because of the social and religious concerns that the blastocyst is destroyed when the ESC lines are generated.
hESC lines can be cultured on feeder cells where they divide infinitely. They can also be grown without feeder cells, where they develop into clusters known as embryoid bodies. Using cells from a human blastocyst in clinical therapy has been difficult, so the 2006 discovery by Yamanaka and Takahashi that pluripotent stem cells could be created from skin cells6 revolutionized the stem cell research. Their pioneering work showed that inducing skin cells with four genes (Oct4, SOX2, Klf4, and c-Myc) would generate pluripotent embryonic stem-like cells in vitro. These cells, known as iPSCs, have the basic properties of hESCs while being derived from somatic cells rather than blastocysts.
An alternative approach for obtaining pluripotent stem cells is somatic cell nuclear transfer (SCNT).13 In this process, the nucleus of an adult human cell is placed in an egg cell that has had its nucleus removed. As this cell divides it can be a source of pluripotent stem cells. A recent study verified that the SCNT pluripotent stem cells are more similar to ESCs than are iPSCs.14 Although much research remains to be done, SCNT pluripotent stem cells appear to have potential in regenerative medicine.
There are many disorders and defects that arise from errors in the complex process of embryonic development. Research over the past decade advanced our understanding of the critical steps in the embryonic development of mice; however, information about the embryonic development of humans remains limited. Although there is overlap with what has been learned from studying mouse embryos, human embryonic growth is different and unique. However, by growing hESCs in the laboratory the multitude of regulatory factors that control the different stages of cell, tissue, and organ system development can be studied and can also provide insights into how our adult tissues are maintained and repaired and allows identification of the causes of birth defects by discovering what interferes with the normal path of cell fate acquisition. The hESCs are also used to produce laboratory disease models in specialized cells like nerve, heart, or beta cells and can also be used for development of new drug therapies.
Despite the much-anticipated potential of hESCs to differentiate and replace malfunctioning cells in the body, progress toward clinical use has been hindered by the possibility of teratoma formation or the immune rejection of the allogeneic transplanted cells and production issues.
Generation of iPSCs has connected several previous observations into a coherent outline. For example, the ability of transcription factor MyoD to change fibroblasts to myoblasts15 and of transcription factor Antennapedia to change development of antennae into legs in Drosophilla,16 uncovered potential of a differentiated cell to assume an alternative cell fate as a result of defined, externally provided signals.
The understanding of induced pluripotency has become more refined as additional reprogramming factors are identified,17 the critical role of epigenetic regulation is uncovered,18 and with the dynamics of iPSC generation (from initially random event to deterministic process) more fully developed.19
The reprogramming technology applied to human cells iPSCs allows for modeling various, typically genetic, disorders.20,21 Furthermore, organoid cultures derived from the patients themselves allow for high throughput drug testing that would be impossible without the supply of differentiated cells from patient-specific iPSCs.
The first preclinical example of iPSC technology conceptually applied to human disease has been amelioration of the sickle cell anemia phenotype in a murine model.22 At the time of this writing, the first iPSC-based clinical trial opened in Japan for individuals with exudative age-related macular degeneration.23
New knowledge derived from the rapidly expanding iPSC field has also reenergized the technology of direct reprogramming, whereby one differentiated cellular phenotype (such as a dermal fibroblast) can be induced to convert into another somatic cell (such as a neuron) without the intermediate iPSC stage. In contrast to the expandable iPSC-based generation of differentiated cells, the process of direct reprogramming makes it more challenging to produce the large numbers of cells needed for therapeutic intervention.
An example of this strategy has been in vivo trans-differentiation of exocrine pancreatic cells or biliary epithelial cells into insulin-producing endocrine cells in rodent models.24,25 A conceptually different concept to solve the same clinical challenge has been blastocyst complementation whereby rat iPSCs were injected into blastocysts that had been derived from mice deficient in pancreatic organogenesis, which resulted in the development of a functional rat pancreas in mice.26 In addition to reducing the cell numbers needed to create a physiologically meaningful effect, the efficacy of both strategies may be enhanced by targeting them into a permissive cellular niche.
The earliest advances with clinical potential will most likely arise from understanding reprogramming in hematopoietic stem cells. Not only was hematopoietic cell transplantation the first stem cell therapy, developed close to half a century ago, but reports of using defined factors to turn committed blood progenitor cells into transplantable hematopoietic cells27,28 suggest that robust generation of clinical-grade, patient-specific autologous grafts for transplantation is possible.
Equally important has been combination of pluripotentiality of ESCs and iPSCs with their commitment to specific lineages, such as hemogenic differentiation program. Derivation of hematopoietic stem cells from murine ESC and their genetic correction has been used in murine severe combined immune deficiency29; similarly in a model of sickle cell anemia, hematopoietic stem cells derived from gene-corrected murine iPSCs22 have established preclinical proof-of-concept for combined gene correction and stem cell engineering. Furthermore, insights from murine embryogenesis were applied to in vitro induction of mesoderm and ESC differentiation to blood cells via coculture with feeder cells or generation of embryoid bodies. These seemingly straightforward concepts, however, have proven challenging to mimic in human ESCs and iPSCs. Despite many attempts, current technology appears to lead only to low hematopoietic chimerism after transplantation of hematopoietic stem cells derived from pluripotent human cells.30,31 An alternative to generation of transplantable human hematopoietic stem cells is direct conversion of fibroblasts to hematopoietic stem cells without the iPSC intermediate. This is done by using forced expression of OCT432 and differentiation of human pluripotent progenitor cells by forced expression of GATA-1, ETV2, and TAL-1 into hemoendothelial cells.33
The replacement of hematopoiesis by marrow transplantation is the prototype of regenerative medicine. While the initial experimentation with marrow transfers on both sides of the Atlantic was almost immediately recognized as a pioneering effort in hematology, it was only later understood as a turning point in the larger field of regenerative medicine. The critical evidence was the ability of a relatively small number of donor cells to repopulate the host and reconstitute its full lymphohematopoietic system. Although initially applied to leukemia and lymphoma therapy in an effort to replace the malignant lymphohematopoiesis with a healthy wild-type system, it later became clear that the immune elimination of the tumor (graft-versus-leukemia, graft-versus-lymphoma) is the dominant mechanism behind successful therapy in many cases.
This remarkable regenerative capacity of hematopoietic stem cells established marrow, and later cord blood, transplantation as the blueprint for other stem cell therapies.
Originally defined by how they were identified—they adhered to the surface of a culture dish34—marrow-derived mesenchymal stromal/stem cells (MSCs) were then identified as key support cells in the cellular niche. Evidence from different sources (e.g., marrow, umbilical cord blood, and adipose tissue) suggests that MSCs have different functions in various organs (e.g., as pericytes in adventitia of blood vessels, or as supporting cells in marrow periosteal and endovascular hematopoietic niches).35,36,37 In addition to this developmental heterogeneity, cultured MSCs display various levels of “stemness,” and the cellular products used in therapeutic applications may be more a cell culture artifact than a counterpart to physiologic functionally integrated MSCs. This is not necessarily a disadvantage, as the culture process enables both amplification of cell numbers and defined release criteria for clinical use.
The most striking application of MSCs in medicine to date relies not on the regenerative capacity of MSCs alone but on the immunosuppressive potential of MSC cultures in the setting of severe graft-versus-host disease (GVHD),38,39,40 a serious complication of allogeneic hematopoietic cell transplantation (HCT).41 The treatment options for individuals with glucocorticoid-resistant severe GVHD have been inadequate, and mortality in this subgroup remains high. In a paradigm-changing study, it was demonstrated that culture-expanded MSCs can ameliorate severe GVHD.38,42 The induction and maintenance of MSC-driven regulation of immune and inflammatory reactions43 has made it possible to assess their role in autoimmune and inflammatory disorders such as Crohn disease, arthritis, diabetes, organ rejection, and bridge therapy before solid-organ transplantation.44,45,46
The regenerative potential of MSCs has long been sought as a tool to rebuild and replace tissues damaged by acute or chronic injury whereby injected cultured MSCs activate endogenous repair mechanisms and disappear in the process.47 In this capacity, MSCs in preclinical models have been shown to alleviate ischemic injury in the heart, brain, and kidneys; toxic insults to lung and liver; and degenerative damage to joints; and it is possible that in some settings allogeneic MSCs may be even more effective than autologous MSCs.48
Lastly, MSCs are relatively easy to gene-modify and thus can be used as cellular vectors for delivering gene therapeutic agents in both inborn genetic disorders and acquired conditions, such as in anticancer therapy or for trophic factor support after surgery.
REGENERATIVE MEDICINE
Cardiovascular disease is a leading cause of death in the world, leading to an estimated 17 million deaths per year.49 As life expectancy in the developed world rises, so too do risk factors associated with chronic heart disease. It is estimated that there are approximately 800,000 new cases of acute myocardial infarction (AMI)50 annually. Heart failure occurs when there is significant deprivation of oxygen to cardiac tissue, which results in decreased cardiac output and function as a result of loss of cardiomyocytes, scar formation, and tissue remodeling.50 Identifying ways to regenerate or repair heart tissue will be key to developing effective treatment options for heart failure.
Since the mid-1990s, scientists have been investigating the potential of adult progenitor cells for use in heart regeneration. These early studies were triggered by the discovery that certain adult tissue-specific stem cells could be differentiated in vitro to become cardiac-like cells.51 This discovery led to many preclinical studies that assessed the ability of adult stem cells to repair or enhance cardiac function after various types of injury.
The cells reported to differentiate in vitro to cardiac-like cells in vivo are satellite cells, which are undifferentiated skeletal muscle myoblasts,52 which led to studies using autologous skeletal myoblasts surgically implanted into the heart muscle.53 Although these cells survived for short periods of time, they retained their intrinsic contractile properties and did not fully integrate into the cardiac tissue,54 which led to arrhythmias and gave little long-term significant benefit in overall heart function.
Some marrow-derived cell populations (lin−; c-kit+) were capable of differentiating to myocytes expressing cardiomyocyte markers such as Nkx2.5, Gata4, and MEF.55 These marrow-derived cells were shown to survive in infarcted hearts and were capable of differentiating into smooth muscle and endothelial cells but not cardiomyocytes in vivo.56 Additional studies demonstrated that other marrow-derived progenitor cell populations (endothelial progenitors, angioblasts, or CD34+ cells) were able to contribute to angiogenesis and neovascularization of the infarcted myocardium.57 This differed from more immature marrow progenitor populations, called side-population cells (Lin− c-kit+ Sca-1+), that not only contribute to neovascularization but also regenerate myocardium.58 These marrow-derived side-population cells homed to the border zone of infarction and resulted in improved left ventricular function.58 The cardiac regeneration capacity of the marrow-derived progenitor cells facilitated large-scale clinical studies using heterogeneous populations of bone mononuclear cells (MNCs), also called epithelial progenitor cells (EPCs), for cardiac repair in patients with AMI59,60 or ischemic cardiomyopathy.61 These studies showed only moderate improvements, and therefore led to further refinement of the selection criteria for marrow-derived cells (CD34+/CD133+) and changes in the route of administration (intracoronary injection) in the Regeneration by Intracoronary Infusion of Selected Population of Stem Cells in Acute Myocardial Infarction (REGENT) study, which still only showed modest success.62
The moderate success of marrow-derived MNCs spurred the investigation of other populations of adult progenitor cells, as well as new routes of administration. The investigations led to the discovery of MSCs, as well as endogenous cardiac-derived stem cells, that could differentiate into cardiomyocytes and endothelial cells in animal models.63 These findings led to clinical studies comparing marrow-derived MNCs versus the new MSCs used for intracoronary injection into patients with ischemic cardiomyopathy.64 These tests showed significant improvement in left ventricle ejection fraction in response to MSC treatment.
To date, the clinical success of cell therapy approaches for cardiac regeneration has been mixed. This is in contrast to the promising early preclinical studies that showed significant improvement in many different measures of cardiac function. This difference has been attributed to the differences between rodent cardiac injury models and human clinical pathology, the cell population administration route, the origin of the cell populations, and the limited number of cells injected.
ESCs, because of their pluripotency and unlimited ability to proliferate, have been the subject of extensive preclinical investigation for many tissues, particularly cardiac tissue repair.65,66 However, there has been less enthusiasm for hESC-derived cardiomyocytes for human cell therapy because their allogeneic nature requires concomitant immunosuppressive therapy and because ethical issues surround their derivation. Despite these challenges, clinical studies have begun to collect ESCs for cardiac differentiation with the intent of being used in a trial for AMI patients. However, as about any somatic cell can be used to generate embryonic stem-like iPSCs,67 with the capability to differentiate into cardiomyocytes, endothelial cells, and smooth muscle cells.68 Initial preclinical studies of murine iPSC-derived cardiomyocytes, injected into ischemic myocardium, led to rejection of transplanted cells by immune reaction as well as continuous proliferation that led to teratoma formation.69 Although iPSCs are attractive for their allogeneic potential they have potential disadvantages for human cell therapy based on their oncogenic nature, epigenetic memory, and maintenance of potency for other cells types.
Growing tissues in vitro for use in regenerative therapies has been investigated as another delivery method of cells for heart repair. This tissue engineering approach involves seeding cells onto scaffolds and growing them for later engraftment or for the generation of whole organs.70 In these systems, cells are transplanted with the scaffolding to the cardiac wall, which provides structural support and a better microenvironment for the migration of cells into the damaged myocardium.71
A common theme in most preclinical and clinical cell therapy-based studies is the demonstration of improvements in cardiac function that is not correlated to number of cells injected or their longevity after administration. This observation has led investigators to speculate that transplanted cells improve cardiac function through paracrine rather than structural effects. This model suggests that observed improvements in myocardial regeneration or vasculogenesis are a result of transplanted cells secreting molecules that are known to improve cardiovascular function after injury.72 The effects of paracrine factors include decreased inflammation, increased angiogenesis, induction of proliferation of cardiomyocytes or protection of existing ones, and activation of endogenous stem cells.73 Ischemic hearts subjected to secreted factors showed an increase in the expression of genes involved in cardiogenesis and a downregulation of cell-death markers, effects that contribute to survival of ischemic cardiomyocytes.74 The advantage of the paracrine model is the potential for the commercial development of paracrine factors that have proven potential for cardiac repair.
The prevalence of lung diseases, like the chronic obstructive pulmonary diseases (COPDs) that include asthma and emphysema, has increased dramatically over the last 50 years. Lung disease is expected to become the third leading cause of disease-related death in the world by 2020. New therapeutic approaches from regenerative medicine are being developed ranging from stem cell therapies to bioengineering of entire tissues of the respiratory system for transplantation. These approaches are based on initial observations that endothelial progenitor cells and mesenchymal stem cells can differentiate in vitro to cells expressing lung epithelial markers and contribute to mature functional bioengineered tissues.
Throughout the pulmonary tract there exist many different niche environments containing distinct epithelial cell types that contribute to the complexity of the lung. Identification of a true endogenous stem cell population that is responsible for maintaining lung tissue under steady state and injury has been challenging and has been a source of controversy.75 Evidence from rodent models and human lungs suggests that the adult endogenous airway, alveolar epithelial cells, lung stroma, and pulmonary vasculature all contain putative stem cell populations that can repair damaged tissue.76,77 These studies suggest the lung has a regional hierarchy of stem and progenitor cells that are specific for proximal versus distal airways as well as alveoli.
Identifying endogenous lung stem cells is complex because many different subpopulations of basal epithelial cells exhibit restricted patterns or roles in self-renewal for steady-state maintenance or after injury.78,79 In the distal airway, putative progenitor cells have been identified in the neuroepithelial body,80 bronchoalveolar duct junction,81 by specific markers of self-renewing lung epithelial cells,82,83 and by function as bronchiolar alveolar stem cells (BASCs). This is in contrast to alveolar epithelial repair thought to be regulated by type 2 alveolar epithelial cells (ATII) because they have been shown to be precursors to type 1 (ATI) cells.84,85 Identification of regionally specific stem cell populations is further complicated by the demonstration that isolated distal airway progenitors (BASCs, CK5+/p63+) can differentiate into ATII and ATI cells.86,87 Regardless, all of these cells show unique functions in repair after injury, reside in different locations in the distal airway and alveolar epithelium, and play different roles as endogenous lung epithelial progenitors.
Many preclinical studies have shown that EPCs can increase function in pulmonary lung injury models.88,89,90 This improvement in function could be because of contributions to structure, paracrine effects, modulation of immune responses, or a combination of these.90 EPCs have also been demonstrated to preferentially home to sites of injury in the lung after systemic administration91; consequently, autologous EPCs have been used clinically in pulmonary hypertension patients and showed improved cardiopulmonary outcomes.92,93
Marrow MSCs are known for their immunomodulatory effects in a wide range of diseases.94,95 The beneficial effect of MSCs results from secretion of soluble mediators and microsomal particles that influence lung progenitor cells directly or indirectly through mediation of inflammatory cells that subsequently promote repair.96,97 Both preclinical and clinical studies have shown efficacy in either systemic or intratracheal administration of MSCs in acute lung injury models, asthma, COPD, and a host of other inflammation-related lung injuries or diseases.75,98 Although different studies have shown varying degrees of efficacy, there are still significant gaps in our understanding of the mechanisms of MSC action on ameliorating disease symptoms and of the specific subtype of MSCs used. This is important, as studies have demonstrated that certain MSCs can have negative effects in some lung disease models, such as pulmonary fibrosis.99,100
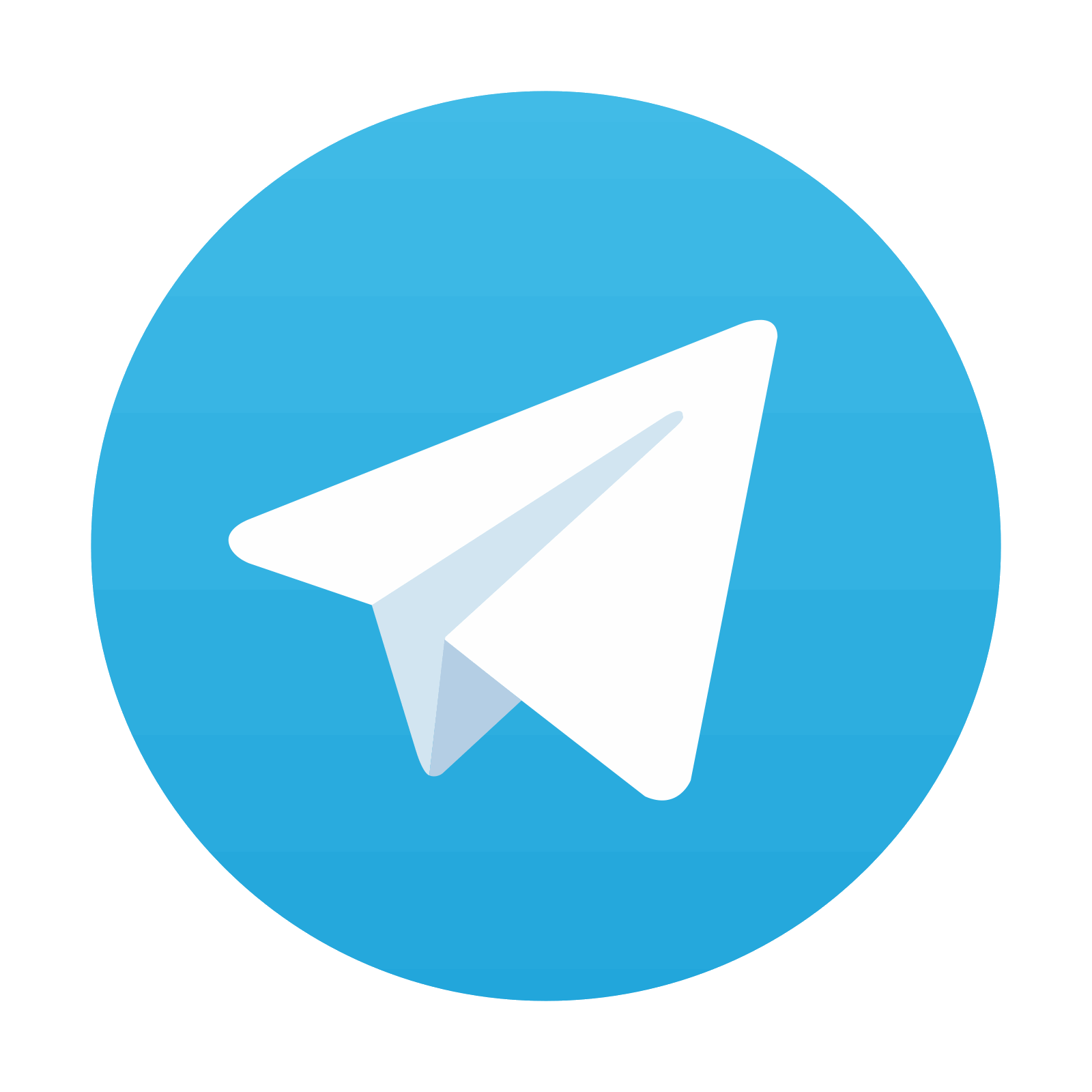
Stay updated, free articles. Join our Telegram channel
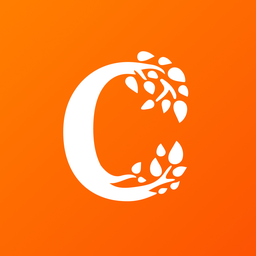
Full access? Get Clinical Tree
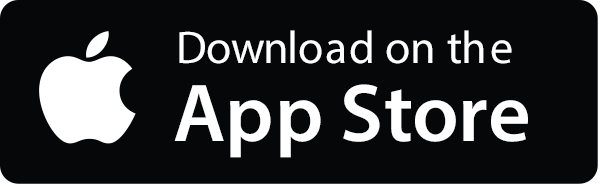
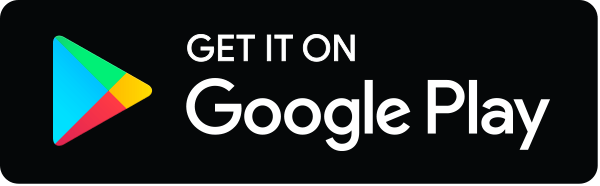