Radiation therapy plays a major role in the treatment of patients with gynecologic malignancies. For women with cervical cancer, radiation therapy is the primary treatment for patients with advanced disease (1,2), yields cure rates equal to those seen after radical surgery for patients with early tumors (3–5), and reduces the risk of local recurrence after surgery for patients with high-risk features (6,7). For women with endometrial cancer, radiation therapy reduces the risk of local recurrence after hysterectomy for patients with high-risk features (8–11) and is a potentially curative primary treatment for patients with recurrent disease and for those who are unfit for primary surgery (12–15). Radiation therapy is an effective treatment for selected patients with ovarian cancer (16,17) and is the primary curative treatment for most patients with invasive vaginal cancer (18,19). It has an expanding role in the management of carcinomas of the vulva (20–22).
Computer technology and information systems have transformed many aspects of radiation therapy practice in the past two decades, making possible three-dimensional treatment planning based on computed tomography (CT) and magnetic resonance imaging (MRI), optimized inverse planning, computer-controlled treatment delivery, and remote afterloading brachytherapy. These techniques enable radiation oncologists to restrict radiation-dose distributions to specified target volumes, thereby delivering the maximal dose to the tumor while sparing normal tissues as much as possible. However, the planning and delivery of these advanced techniques are labor-intensive, costly, and potentially more prone to error than the planning and delivery of traditional, generally simpler techniques.
Radiation biologists and clinicians continue to advance our understanding of the molecular mechanisms involved in radiation-induced cell death, the nature of drug–radiation interactions, and the importance of radiation dose, the time over which the dose is given, and the dose per fraction. In 1999 and 2000, the results of randomized clinical trials demonstrated a significant improvement in pelvic disease control and survival when concurrent chemotherapy was added to radiation therapy for patients with locally advanced cervical cancer (20–22). These results led to one of the most significant changes in the standard treatment of gynecologic cancers in decades.
In this chapter, the basic principles of radiation therapy, radiation biology, and radiation physics are reviewed, and an overview of the indications for, and techniques of, radiation therapy in the treatment of gynecologic malignancies is presented.
Radiation Biology
Radiation Damage and Repair
Cell death can be defined as the loss of clonogenic capacity (i.e., the ability of the cell to reproduce). Most cell death caused by ionizing radiation is mitotic cell death. Ionizing radiation may also cause programmed cell death (apoptosis).
The critical target for most radiation-induced cell death is the DNA within the cell’s nucleus. Photons or charged particles interact with intracellular water to produce highly reactive free radicals that in turn interact with DNA to produce strand breaks that interfere with the cell’s ability to reproduce. Although this interaction may cause a cell’s “reproductive death,” the cell may continue to be metabolically alive for some time. Radiation-induced damage may not be expressed morphologically until days or months later when the cell attempts to divide (mitotic cell death). In some cases, a damaged cell may undergo a limited number of divisions before it dies, having lost the ability to reproduce indefinitely.
Apoptosis (programmed cell death) may play an important role in radiation-induced cell death (23). In contrast to mitotic cell death, apoptosis may occur before cell division or after the cell has completed mitosis. The plasma membrane and nuclear DNA may both be important targets for this type of cell death. Apoptosis appears to be a particularly important mechanism of radiation-induced cell death in certain postmitotic normal tissues, including human salivary glands and lymphocytes. Radiation-induced apoptosis has been observed in some proliferating normal tissues and tumors. Biologists are actively studying the pathways that regulate the expression of radiation-induced apoptosis, in the hope that they can be exploited to improve local tumor control.
Cell Survival Curves
The effects of ionizing radiation on the survival of mammalian cell populations in vitro are typically expressed graphically as dose–response or “cell survival” curves (24). The surviving fraction of cells is plotted (on an exponential scale) against the dose of radiation (on a linear scale). Experimental data using single doses of sparsely ionizing radiation (e.g., x-rays, gamma rays, electrons, or protons) typically produce cell survival curves with two components (Fig. 4.1): A shoulder region and an exponential region.
Several mathematical models, based on different hypothetical mechanisms of cell killing, have been devised to describe radiation dose–response relationships. These include the following:
1. The multitarget model (also referred to as the N-D0 model).
2. The linear-quadratic model (also referred to as the α/β model).
The multitarget model (Fig. 4.1A) is described by the expression logeN = Dq/D0, where N and Dq measure the width of the shoulder and D0 is the slope of the final exponential portion of the survival curve. This model derives from the classic target theory, which holds that each cell contains multiple sensitive targets, all of which must be hit to kill the cell. The presence of a shoulder region is believed to reflect accumulation of sublethal injury in some of the irradiated cells (24,25). Although the multitarget model accurately describes the exponential portion of the dose–response curve, it is a poor fit to experimental data in the shoulder region. In particular, it fails to predict the approximately linear slope (D1) of the initial portion of the shoulder (Fig. 4.1B).
The linear-quadratic model describes the dose–response relationship according to the equation S = exp − (αD + βD2), where S is the surviving fraction, D is the dose of radiation, and α and β are constants (Fig. 4.1B). This model presupposes two components of cell death: one that is proportional to the dose (αD) and one that is proportional to the square of the dose (βD2). The dose at which the linear and quadratic components are equal is α/β (Fig. 4.1A). This model fits experimental data particularly well for the first few logs of cell death, which are most relevant to fractionated and low-dose-rate (LDR) irradiation, but it is continuously bending on a log-linear plot. This bend is inconsistent with experimental data that demonstrate a straight line on a log-linear plot for the distal portion of the cell survival curve. For this reason, the linear-quadratic model may not accurately predict the effect of treatment schedules that involve very large doses per radiation fraction.
Figure 4.1 Parameters commonly used to characterize the relationship between radiation dose and cell survival in mammalian culture. In the multitarget, or N-D0, model (A), N is the extrapolation number, N and Dq measure the width of the shoulder, and D0 represents the slope of the final exponential portion of the survival curve. The multitarget model provides an accurate description of experimental data in the exponential portion of the survival curve. The linear-quadratic model (B) more accurately describes the shape of the initial shoulder portion of the curve. Because the shoulder has more influence on fractionated radiation therapy, the linear-quadratic model is more often used to predict the results of fractionated clinical radiation therapy. (Modified from Hall EJ. Radiobiology for the Radiologist. 5th ed. Philadelphia, PA: Lippincott Williams & Wilkins; 2000, with permission.)
Fractionation
Conventional radiation therapy is usually given in a fractionated course with daily doses of 180 to 200 cGy (centiGray) per fraction. Hypothetical cell survival curves for normal tissue and tumor cells illustrate the advantage of fractionation (Fig. 4.2). When a dose of radiation is divided into multiple smaller doses separated by an interval sufficient to allow maximum repair of sublethal injury, a relatively shallow dose–response curve is achieved, reflecting a repetition of the shoulder of the single-dose cell survival curve. The slope of the fractionated-dose cell survival curve depends on the character of the shoulder (N and Dq). The sparing effect of fractionation is greatest for cells with a response to radiation characterized by a relatively broad shoulder, reflecting the cells’ greater ability to accumulate and repair sublethal damage during the interfraction interval. Many normal tissues and some poorly responsive tumors exhibit this type of response to fractionated irradiation in vivo and in vitro. In contrast, most tumors and some acutely responding normal tissues (e.g., bone marrow and intestinal crypt cells) have a dose–response curve with a relatively narrow shoulder, implying relatively little sparing effect of fractionation.
The biologic effects of various fractionation schemes can be estimated and compared using the linear-quadratic formula. For a total radiation dose d divided in n well-separated fractions, the biologic effect is given by E = n (αd + βd2). Some rearrangement of the equation gives: E/α = nd × (1 + d/(α/β)). The quantity E/α is termed the biologically effective dose (BED) and is the value used to compare various fractionation schedules. The value of α/β provides an estimate of the fractionation sensitivity of a tissue. Tissues that are greatly spared by fractionation (most normal tissues) generally have a low α/β value, while tumors and acutely responding tissues tend to have a relatively high value.
The difference between the fractionation sensitivity of tumors and normal tissues is an important determinant of the therapeutic ratio (the difference between tumor control and normal tissue complications) of fractionated irradiation.
Dose-rate Effect
So far, this discussion of cell survival curves and fractionation has referred to radiation given in acute exposures—that is, at a rate of 100 cGy per minute or greater. At these dose rates, the shoulder of the survival curve is pronounced. As the dose rate is decreased, cells have a greater opportunity to repair sublethal injury during the exposure. This is called the dose-rate effect. The slope of the survival curve becomes increasingly shallow and the shoulder less apparent (Fig. 4.3) until a dose rate is reached at which all sublethal injury is repaired. In experimental systems, the dose-rate effect appears to be much more pronounced for normal cells than for tumor cells. This differential effect implies a favorable therapeutic ratio that is exploited with LDR intracavitary and interstitial brachytherapy.
The Four Rs
The biologic effect of a given dose of radiation is influenced by the dose, fraction size, interfraction interval, and time over which the dose is given. Four factors, classically referred to as “the four Rs of radiobiology,” govern the influence of dose, time, and fractionation on the cellular response to radiation. These are the following:
1. Repair
2. Repopulation
3. Redistribution
4. Reoxygenation
Repair
Because fractionated irradiation permits greater recovery of sublethal injury during treatment, a higher total dose of radiation is required to achieve a given biologic effect when the total dose is divided into smaller fractions. The broader the shoulder of the survival curve, the greater the increase in dose required to achieve the same level of cell death as achieved by a single dose. Two-dose experiments with varying interfraction intervals have indicated that a space of at least 4 hours, and probably more than 6 hours, is necessary to complete repair of accumulated sublethal injury. Clinical studies tend to confirm these findings; for this reason, altered-fractionation protocols usually require a minimum interval of 4 to 6 hours between treatments.
Figure 4.2 Relationship between radiation dose and surviving fraction of cells treated in vitro with radiation delivered in a single dose or in fractions. Top: Most tumors and acutely responding normal tissues. Bottom: Late-responding normal tissues. For most tumors and acutely responding normal tissues, the cellular response to single doses of radiation is described by a curve with a relatively shallow initial shoulder (Top, yellow line). Cellular survival curves for late-responding normal tissues (Bottom, yellow line) have a more pronounced shoulder, suggesting that these cells have a greater capacity to accumulate and repair sublethal radiation injury. When the total dose of radiation is delivered in several smaller fractions (Dose A [dose/fraction] = blue line, or a larger fraction Dose B [dose/fraction] = red line), the response to each fraction is similar and the overall radiation survival curve reflects multiple repetitions of the initial portion of the single-dose survival curve. Note that the total dose required to kill a specific proportion of the cells decreases as the dose per fraction increases (red line). Arrows indicate the differential effects of relatively large versus small fractions of radiation. The greater differential effects of fractionated irradiation on normal tissues (Bottom) than on tumor (Top) reflect the greater capacity of late-responding normal tissues to accumulate and repair sublethal radiation injury. (From Karcher KH, Kogelnik HD, Reinartz G, eds. Progress in Radio-Oncology II. New York, NY: Raven Press; 1982:287–296.)
Figure 4.3 Response of mouse jejunal crypt cells to different dose rates of γ rays. The mice were subjected to total body irradiation, and the proportion of surviving crypt cells was determined by counting regenerating microcolonies in the crypts 3.5 days after irradiation. There was a dramatic difference in cell killing because of repair of sublethal injury at low dose rates. In this system, the lowest dose rate (0.54 cGy per minute) causes little reduction in the number of surviving cells even after high doses because repopulation during the long exposure balances the cell killing from radiation. (From Fu KK, Phillips TL, Kane LJ, et al. Tumor and normal tissue response to irradiation in vivo: Variation with decreasing dose rates. Radiology. 1975;114:709–716, with permission.)
Repopulation
Repopulation refers to the cell proliferation that occurs during the delivery of radiation. The magnitude of the effect of repopulation on the dose required to produce a given level of cell death depends on the doubling time of the cells involved. For cells with a relatively short doubling time, a significant increase in dose may be required to compensate for a protraction in the delivery time. This phenomenon may be of considerable practical importance. The speed of repopulation of normal tissues that manifest radiation injury soon after exposure (skin, mucosal surfaces, etc.) limits contraction of a course of fractionated irradiation. Unnecessary protraction probably reduces the effectiveness of a dose of radiation by permitting time for repopulation of malignant clonogens during treatment (26,27). Cytotoxic treatments—including chemotherapy, radiation therapy, and possibly surgical resection—may trigger an increase in the proliferation rate of surviving clonogens. This accelerated repopulation may increase the detrimental effect of treatment delays and may influence the effectiveness of sequential multimodality treatments (28,29).
Redistribution
Studies of synchronized cell populations show significant differences in the radiosensitivity of cells in different phases of the cell cycle (30). Cells are usually most sensitive to radiation in the late G2 phase and during mitosis and are most resistant in the mid- to late S and early G1 phases. When asynchronous dividing cells receive a fractionated dose of radiation, the first fraction tends to synchronize the cells by killing off those in sensitive phases of the cell cycle. Cells remaining in the S phase begin to progress to a more sensitive phase of the cell cycle during the interval before the next fraction is given. This redistribution of cells to a more sensitive phase of the cell cycle tends to increase the overall cell death achieved from a fractionated dose of ionizing radiation, particularly if the cells have a relatively short cell cycle time.
Reoxygenation
The sensitivity of fully oxygenated cells to sparsely ionizing radiation is approximately three times that of cells irradiated under anoxic conditions. This makes oxygen the most effective known radiation sensitizer. The molecular interactions responsible for the oxygen effect are not completely understood, but it is believed that oxygen stabilizes the reactive free radicals produced by the ionizing events. The ratio between the dose needed to achieve a given level of cell death under oxygenated versus hypoxic conditions is referred to as the oxygen enhancement ratio (Fig. 4.4).
Figure 4.4 Survival curves for mammalian cells irradiated under aerated and hypoxic conditions. The dose required to produce a given level of damage is approximately three times greater under hypoxic or anoxic conditions than under fully oxygenated conditions. The ratio of doses is the oxygen enhancement ratio (OER). Sometimes the shoulder also is reduced under hypoxic conditions. (Modified from Hall EJ. Radiobiology for the Radiologist. 5th ed. Philadelphia, PA: Lippincott Williams & Wilkins; 2000, with permission.)
Most normal tissues are fully oxygenated, but significant hypoxia occurs in at least some solid tumors, rendering the tumor cells relatively resistant to the effects of radiation. The clinical importance of tumor hypoxia is uncertain, because hypoxic cells initially tend to become better oxygenated during a course of fractionated irradiation (31). This phenomenon, called reoxygenation, tends to increase the response of tumors to a dose of fractionated radiation.
Treatment Strategies for Overcoming Radioresistance of Hypoxic Cells
Many treatment strategies have been explored to overcome the relative radioresistance of hypoxic cells in human solid tumors (32–37). These include the following:
1. Hyperbaric oxygen or carbogen breathing
2. Red cell transfusion or use of growth factors
3. Pharmacologic agents that act as hypoxic cell sensitizers (e.g., misonidazole) or that selectively kill potentially radioresistant hypoxic cells (e.g., tirapazamine)
4. High-linear-energy-transfer radiation
None of these approaches have clearly demonstrated an improvement in outcome; however, many of the relevant studies were severely compromised by technical or logistical problems.
Numerous retrospective studies have found a correlation between the minimum hemoglobin level during treatment and outcome, but all of them were compromised by possible confounding risk factors (38–40). Even with multivariate analysis, investigators have been unable to sort out whether anemic patients have poorer responses to radiation because of their low hemoglobin levels or have low hemoglobin levels because poorly responsive tumors are more likely to bleed. Studies of intratumoral oxygen tension have suggested that patients with hypoxic tumors tend to have a poor prognosis; this correlation appears to be present in surgically treated patients, and may in part reflect a tendency for biologically aggressive tumors to be hypoxic (41).
An early randomized study of transfusion in anemic patients with locally advanced cervical cancer hinted at improved local control when oxygen-carrying capacity was increased (41). The findings of this small study have not yet been confirmed in a larger prospective trial, and the results remain inconclusive. One group of investigators (42) suggested that allogeneic transfusion may be harmful, although their results conflict with those of most other studies. Nevertheless, tumor hypoxia continues to be one probable cause of the failure of irradiation to control some tumors (e.g., advanced cervical cancers with a significant population of hypoxic tumor cells), and most clinicians recommend that the hemoglobin level be maintained above 10 g/dL during radiation therapy (43).
In the late 1990s, the commercial availability of recombinant erythropoietin led investigators to explore the impact of growth factor–induced increases in the hemoglobin level on the outcome of patients treated with radiation therapy. Initial enthusiasm was tempered by negative results of a randomized trial in patients with head and neck cancer and by reports of increased thromboembolic events in patients receiving erythropoietin (44). The Gynecologic Oncology Group prematurely closed a randomized trial of chemoradiation with or without erythropoietin in patients with locally advanced cervical cancer because of concerns about the risk of thromboembolism (45). In that study, thrombotic events occurred in 11 of 57 patients (19%) who received erythropoietin versus 4 of 52 (8%) treated with chemoradiation alone (p = NS); the impact of erythropoietin on outcome was inconclusive because of the small number of patients in the study.
Linear-energy Transfer and Relative Biologic Effectiveness
The rate of deposition of energy along the path of the radiation beam is referred to as its linear-energy transfer (46). Photons, high-energy electrons, and protons produce sparsely ionizing radiation beams (low-linear-energy transfer), whereas larger atomic particles (e.g., neutrons, alpha particles, and carbon ions) produce much more densely ionizing radiation beams (high-linear-energy transfer). The biologic effects of densely ionizing radiation beams differ in several important ways from those of more sparsely ionizing radiation beams. With high-linear-energy-transfer radiation beams:
1. There is little or no repairable injury and therefore no shoulder on the tumor cell survival curve.
2. The magnitude of cell death from a given dose is greater, increasing the terminal slope of the survival curve.
3. The oxygen enhancement ratio is diminished.
The unit of relative biologic effectiveness is used to compare the effects of different radiation beams. Relative biologic effectiveness is defined as the ratio between a test radiation dose and the dose of 250-kV x-rays needed to produce a specific biologic effect. The relative biologic effectiveness may differ somewhat according to the tissue and biologic end point being studied.
In practice, few facilities exist for the production of high-linear-energy-transfer beams, and their use has had no major impact on the results of treatment for gynecologic malignancies.
Hyperthermia
Temperature is another factor that can modify the effect of ionizing radiation (24). Supraphysiologic temperatures alone can be toxic to cells because heat is preferentially toxic to cells in a low-pH environment (frequent in areas of hypoxia) and to cells in the relatively radioresistant S phase of the cell cycle. Temperatures in the range of 42°C to 43°C sensitize cells to radiation by reducing the shoulder and increasing the slope of the cell survival curve. Because of the different vascular supplies of tumors and normal tissues, hyperthermia may produce greater temperature elevations in tumors, increasing the possible therapeutic advantage when heat is combined with irradiation. Biologists and clinicians tried to find ways to exploit this effect for many years but were hampered by technologic limitations on the ability to selectively heat deep-seated tumors (47). A trial from Amsterdam (48) reported that survival was improved when hyperthermia was used with irradiation in patients with locally advanced cervical cancer. The patients in this study received relatively low doses of radiation, did not receive concurrent chemotherapy, and had poorer than expected pelvic disease control in the control arm, but the findings suggest that the approach may deserve further study.
Interactions between Radiation and Drugs
Drugs and radiation interact in a number of ways to modify cellular responses. Steel and Peckham (49) categorized these interactions into four groups: Spatial cooperation (independent action), additivity, supra-additivity, and subadditivity.
Spatial Cooperation— Independent Action
Spatial cooperation is the situation in which drugs and radiation act independently with different targets and mechanisms of action so that the total effect of the combination is equal to that of each agent separately. For example, a site that is protected from chemotherapy (e.g., the brain) may be treated with radiation to prevent recurrence. Alternatively, a drug may be used to destroy microscopic distant disease, while radiation is used to sterilize local tumor.
Additivity
Additivity is the situation in which two agents act on the same target to cause damage that is equal to the sum of their individual toxic effects.
Supra-additivity
When there is supra-additivity, a drug potentiates the effect of radiation, causing a greater response than would be expected from simple additivity.
Subadditivity
With subadditivity, the amount of cell death that results from the use of the two agents is less than that expected from simple additivity (the amount may still be greater than expected from either treatment alone).
Clinically, it is difficult to determine which mode of interaction occurs when two agents are used concurrently. When a greater response is observed than would be expected from radiation alone, the interaction is often described as synergistic, but may be only additive or even subadditive.
Therapeutic Ratio
Ionizing radiation interacts with all the tissues in its path, not exclusively tumor tissue. Radiation can be considered an effective cancer treatment only if there is a differential biologic effect on tumor and normal tissues. The difference between tumor control and normal tissue complications is referred to as the therapeutic gain or therapeutic ratio.
Figure 4.5 Theoretical sigmoid dose–response curves for tumor control and severe complications. The therapeutic ratio is related to the distance between the two curves. Dose A controls tumor in 80% of cases with a 5% incidence of complications (blue curve). Dose B yields a 10–15% increase in the tumor control probability but a much greater risk of complications, narrowing the therapeutic ratio (orange curve).
In general, the relationship between the probability of tumor cure or the probability of normal tissue injury and the dose of radiation can be described by a sigmoid curve (Fig. 4.5). At relatively low radiation doses, there is an insufficient amount of cell death to produce any likelihood of tumor cure. As the dose is increased, a threshold is reached at which some cures begin to be observed. For most tumor systems, the likelihood of cure rises rapidly as the radiation dose is increased beyond this threshold and then reaches a plateau. The shape and slope of the dose–response curve vary according to the tumor type and size (50,51).
A similar sigmoid relationship is seen when the likelihood of complications is plotted against the radiation dose. If the sigmoid curve for normal tissue complications is to the right of the sigmoid curve for tumor control, then treatment with doses that fall between the two curves may achieve tumor control without causing complications. The difference between these curves represents the therapeutic ratio. The primary goal of radiation research efforts is to improve the therapeutic ratio by increasing the separation between these dose–response curves, maximizing the probability of complication-free tumor control.
Effects of Radiation on Normal Tissues
The extent of radiation damage to normal tissues depends on a number of factors, including the radiation dose, the organ, the volume of tissue irradiated, and the division rate of the irradiated cells. Tissues that have rapid cell turnover (i.e., tissues whose functional activity requires constant cell renewal) tend to manifest radiation injury soon after exposure, often during a fractionated course of radiation therapy. Examples of acutely responding tissues include most epithelia (e.g., skin, hair, gastrointestinal mucosa, bone marrow, and reproductive tissues). In contrast, tissues that have slower cell turnover (i.e., tissues whose functional activity does not require constant cell renewal) tend to manifest radiation injury months or years after exposure to radiation. Examples of late-responding tissues are the connective tissues, muscle, and neural tissues.
In some normal tissues, cell death may occur through the mechanism of apoptosis. Although apoptosis is not the primary mechanism of damage in most normal tissue injury, it is important in the response of lymphocytes, salivary gland cells, and a small proportion of intestinal crypt cells (23).
Acute Reactions
Acute reactions to pelvic irradiation, such as diarrhea, are usually associated with mucosal denudation, which in turn stimulates an increase in cell proliferation (52). This regenerative response is usually sufficient to prevent serious side effects with weekly doses of 900 to 1,000 cGy given in five fractions. This empirically derived schedule is the most commonly used for clinical radiation therapy. If treatment is accelerated to deliver the dose over much shorter periods, then the regenerative capacity of the epithelium may be overwhelmed and the acute reaction so severe that a break in treatment is needed to allow for epithelial regeneration. The severity of acute reactions depends on the volume of the normal tissues irradiated and the specific nature of the tissues.
Late Reactions
The pathogenesis of late radiation complications (i.e., those that occur months to years after radiation therapy) differs from that of acute reactions and is still incompletely understood. It is hypothesized that late effects of radiation result from the following:
1. Damage to vascular stroma that causes an epithelial proliferation with decreased blood supply and subsequent fibrosis.
2. Damage to slowly or infrequently proliferating parenchymal stem cells that eventually results in loss of tissue or organ function (52).
The likelihood of developing serious late effects from radiation depends on many factors, including, but not limited to, the dose of radiation, the radiation dose per fraction, the volume of tissue irradiated, the radiation dose-rate, patient characteristics, other treatments (such as surgery or chemotherapy), and the end point being measured.
Because late-responding tissues are not proliferating rapidly, the duration of a course of radiation treatment does not alter their tolerance. Late-responding normal tissues tend to be quite sensitive to changes in the dose per fraction so that for a given dose of radiation administered over a given period, the risk of late effects will be greater with larger fractions. This fractionation effect is responsible for the advantage of altered fractionation schedules in clinical settings in which late normal tissue reactions are severely dose limiting (Fig. 4.2) (53–55). This fractionation effect has important implications for treatments such as high-dose-rate (HDR) brachytherapy, intensity-modulated radiation therapy (IMRT), and stereotactic body radiation therapy (SBRT) where a portion of the target (and possibly adjacent normal tissues) frequently receives doses of more than 2 Gy per fraction.
Some tissues—such as the liver, kidney, and lung—consist of functional subunits that are arranged more or less in parallel; these tissues can tolerate a high dose of radiation given to a small portion of the organ without serious late effects, but tend to be relatively sensitive to moderate whole-organ doses. Other organs, such as bowel or ureter, are organized in a serial fashion—delivery of a damaging dose to even a small portion of the organ can cause total organ failure. For all of the reasons discussed previously, normal tissue tolerances cannot be described in terms of simple dose limits. Some generalizations can be made about the tolerance of individual tissues (doses refer to external radiation given in daily fractions of 1.8 to 2 Gy or with LDR brachytherapy).
Uterus The uterus and cervix are typically described as resistant to radiation; however, what is really meant by this is that the uterus can be treated to very high doses (more than 100 Gy in some cases) without the patient developing serious complications in adjacent critical structures (e.g., bowel and bladder). The uterus probably cannot sustain pregnancy after such doses. Even moderate doses of 40 to 50 Gy probably cause enough smooth muscle atrophy to prohibit successful term pregnancy, but this is rarely tested. Women who have received 20 to 30 Gy or more to the uterus during the perimenarchal period have become pregnant, but have tended to have spontaneous second trimester abortions, probably because of underdevelopment of the uterus. Patches of endometrium frequently continue to function after doses of 50 Gy or more.
Ovary The radiation dose required to cause ovarian failure is highly dependent on the patient’s age. Perimenarchal girls may continue to menstruate and can become pregnant after receiving as much as 30 Gy to the ovaries; however, they usually experience premature menopause 10 to 20 years later. Most adult women have ovarian failure after 20 Gy; as little as 5 to 10 Gy can induce menopause in older premenopausal women.
Vagina The radiation tolerance of the vagina depends on the region (upper, middle, lower, anterior, posterior, or lateral), length of vagina treated and radiation dose, fraction size, dose rate, hormonal support, and other factors. Small portions of the surface of the lateral apical vagina can be treated to a very high dose (ê140 Gy) without causing major complications in adjacent structures. However, these high doses cause atrophy and shortening of the apical vagina. The vaginal tolerance dose is less if treatment includes more than the apical vagina, or if the dose includes the posterior, or distal, vagina. Even moderate doses (40 to 50 Gy) may decrease the elasticity of the vagina, although it is sometimes difficult to distinguish the direct effects of radiation from those of tumor, altered hormonal environment, aging, and other factors.
Small Intestine The risk of small intestinal side effects is highly dependent on the radiation dose and volume irradiated and on the patient’s history. In the absence of complicating factors, the entire small intestine can tolerate doses up to 30 Gy without major late effects. Smaller volumes can tolerate 45 to 50 Gy with a low risk of complications; the risk of chronic diarrhea and bowel obstruction increases rapidly with doses greater than 50 to 60 Gy and approaches 100% if a significant volume of small bowel receives 70 Gy or more. The risk of bowel obstruction is significantly increased in patients who have a history of major transperitoneal surgery, pelvic infection, or heavy smoking (56).
Rectum In most cases, the entire rectum can tolerate 45 to 50 Gy with a low risk of major sequelae. Small portions of the anterior rectal wall can tolerate doses of at least 70 to 75 Gy. The risk of serious late effects (severe bleeding, obstruction, or fistula) increases steeply as the volume of rectum treated to high dose is increased.
Bladder The entire bladder can be treated to 45 to 50 Gy with a very low rate of serious morbidity. This dose may have subtle effects on bladder contractility, particularly in patients who have undergone radical hysterectomy. Small portions of the bladder can tolerate doses of 80 Gy or more with a low risk of major morbidity (severe bleeding, contracture, or fistula). The dose–response relationship is poorly defined in this range, because traditional methods of bladder dose estimation have used reference points that systematically underestimated the maximum dose. Newer CT- and MRI-based treatment planning methods yield more accurate estimates that should provide the basis for a better understanding of bladder tolerance doses in the future.
Ureter Surgically undisturbed ureters appear to tolerate 85 to 90 Gy of combined external beam radiation and LDR intracavitary treatment with a low risk of stricture.
Kidney Most patients can tolerate as much as 18 to 22 Gy to both kidneys with very little risk of long-term damage. Higher doses cause permanent damage to renal parenchyma. If the patient has normal renal function, 50% or more of the renal parenchyma can be treated to a high dose without causing renal failure; however, renal hypertension may occur if an entire kidney is obliterated with radiation. Underlying renal disease or concurrent use of chemotherapy can decrease renal tolerance.
Liver In most cases, the liver can tolerate as much as 30 Gy (at 1.5 Gy per fraction) to the entire organ, although this dose will cause transient elevation of alkaline phosphatase levels and can cause dysfunction in a small proportion of patients. Higher doses cause serious damage to liver parenchyma but can be tolerated if delivered to only a portion of the liver. Tolerance is highly dependent on underlying hepatic function and can be markedly decreased with concurrent delivery of some chemotherapeutic agents and during periods of hepatocyte regeneration (e.g., after partial hepatectomy).
Spinal Cord and Nerves Transverse myelitis and paralysis can occur in a small proportion of patients who receive doses as low as 50 Gy to the spinal cord, and the risk increases rapidly as the dose approaches 60 Gy at 2 Gy per fraction. Peripheral nerves, including the cauda equina, are rarely affected after 50 Gy and usually tolerate doses as high as 60 Gy without serious sequelae.
Bone As little as 10 to 15 Gy of radiation causes transient depletion of bone marrow elements. With doses of more than 30 to 40 Gy, permanent damage is done to supporting elements, and bone marrow within the irradiated area will not repopulate normally. This damage can be seen as fatty replacement of the marrow cavity on MRI. The risk of fracture after radiation therapy depends on the bone irradiated, the volume of bone in the high-dose region, bone density, concomitant steroid use, and other factors.
Symptomatic fracture is rare after treatment with 40 to 45 Gy of pelvic radiation. However, routine MRI sometimes detects small, usually asymptomatic, insufficiency fractures of the pelvis after this dose (57). Hip fracture may be seen after doses as low as 40 Gy to the entire femoral head and neck, and the risk probably increases rapidly as the dose approaches 60 Gy.
Treatment Strategies to Exploit Differences between Tumor and Normal Tissue in the Response to Fractionated Radiation Therapy
A variety of altered fractionation schemes have been devised to exploit the different sensitivities of tumor and normal tissues to fractionation and the possible effects of tumor cell repopulation. These include hyperfractionation, in which the dose per fraction is reduced, the number of fractions and total dose are increased, and the overall treatment time is relatively unchanged; accelerated fractionation, in which the dose per fraction is unchanged, the overall treatment duration is reduced, and the total dose is unchanged or decreased; and hypofractionation, in which the dose per fraction is increased, the number of fractions and total dose are reduced, and the overall treatment time is decreased.
With hyperfractionation, treatment is usually given two or more times daily with at least 4 to 6 hours between fractions to allow repair of sublethal injury. This scheme should permit delivery of a higher dose of radiation without increasing the risk of late complications or the overall duration of treatment. Hyperfractionation schemes may have an advantage if the increased dose delivered per day does not cause unacceptable acute effects and if patients are willing to accept the added inconvenience of two or three treatments daily.
Accelerated fractionation schemes do not reduce the risk of late effects and tend to increase the acute effects of treatment, but may be advantageous because treatment is completed over a shorter time, reducing tumor cell repopulation during treatment (55). Such schemes are likely to be of limited value in the management of gynecologic malignancies, because acute side effects tend to limit the rate of treatment delivery.
Hypofractionation schedules are usually avoided when treatment is likely to cure the patient, because the α/β of late-responding normal tissues is less than the α/β of most tumors, meaning that large fractions have a therapeutic disadvantage. Malignant melanoma, which appears to have a relatively low α/β, may be a rare exception to this pattern. Hypofractionated schedules are frequently used for palliative treatment because they are convenient and produce rapid symptom relief. However, the necessary reduction in dose reduces the likelihood of complete eradication of tumor within the treatment field. Hypofractionation may be particularly beneficial if the tumor target is some distance from critical structures and if the radiation treatment plan is characterized by a steep dose gradient such that the target receives a relatively high dose per fraction, while normal tissue structures receive no more than approximately 2 Gy per fraction. Under ideal circumstances, HDR brachytherapy plans and some highly conformal external beam plans achieve this favorable geometry.
SBRT, sometimes referred to as stereotactic radiosurgery, is an extreme form of hypofractionation in which a small number of large doses of radiation (usually five or fewer) are used to ablate tumor. Because there is very little sublethal injury with these schedules, tight geometrical conformality is needed to obtain a favorable balance between tumor control and normal tissue preservation. For this reason, great care must be taken to avoid exposing vulnerable normal tissues to the target dose.
Treatment is usually delivered using highly conformal treatment plans with precise patient positioning and immobilization. These techniques are particularly useful for treatment of tumors surrounded by normal tissues that have a parallel structure (e.g., lung or liver) because ablation of a small volume of these normal tissues has little effect on the overall organ function. SBRT may be more dangerous in situations where tumor is very close to serially structured organs, which can be seriously compromised if even a small portion of the organ is severely damaged (e.g., bowel, ureter, or bladder).
For this reason, SBRT should be used with extreme caution in the pelvis, where tumors are typically surrounded by vulnerable structures and where very tightly conforming treatment plans may lead to undertreatment of a portion of the tumor.
Combinations of Surgery and Radiation Therapy
Because surgery and radiation therapy are both effective treatments, clinicians have tried to improve locoregional control or reduce treatment morbidity by combining the two modalities. Theoretically, surgery may remove bulky tumor that may be difficult to control with tolerable doses of radiation, and radiation may sterilize microscopic disease at the periphery of the surgical bed. The two modalities are combined in a number of ways:
1. Preoperative irradiation
2. Diagnostic surgery (surgical staging) followed by definitive irradiation
3. Intraoperative irradiation
4. Surgical resection followed by postoperative irradiation
5. Combinations of these approaches
Preoperative Irradiation
Preoperative irradiation is sometimes used to sterilize possible microscopic disease at the margins of a planned operative site. This is potentially most useful when the surgeon anticipates close margins adjacent to a critical structure (e.g., the urethra or anus in a patient with locally advanced vulvar cancer).
In patients with uterine cancer, preoperative irradiation has largely been abandoned in favor of postoperative irradiation, which can be planned when information from the surgical specimen is available and which avoids unnecessarily treating patients with very-early-stage disease. Preoperative irradiation is sometimes used to treat patients with stage II endometrial cancer that grossly involves the cervix, and is used in some patients with bulky cervical cancers. This is because the dose deliverable to paravaginal tissues is much greater when the uterus is still in place to hold an intrauterine applicator than after surgery, when only an intravaginal applicator can be used.
Some studies suggested that lower doses of radiation may be required to sterilize microscopic disease in a tumor bed undisturbed by surgery because an intact vascular supply is better able to deliver oxygen. Because the risk of operative complications is increased after high-dose radiation therapy, doses given when surgical resection is anticipated are usually lower than doses given when a tumor is irradiated definitively. The greatest risk of preoperative radiation therapy is that if the tumor remains unresectable, the effectiveness of additional irradiation will be markedly decreased by the long interval between treatments.
Intraoperative Irradiation
In some cases, intraoperative irradiation can be delivered with a permanent implant (using 125I or 198Au), with afterloading catheters in the operative bed (using 192Ir), or with a special electron beam or orthovoltage unit in the operating room. These approaches deliver radiation directly to the site of maximum risk when the target can be visualized directly and normal tissues nearest the treatment area can be removed from the radiation field. Removal of normal tissues from the treatment field is an important physical advantage of intraoperative external beam techniques that may counterbalance the biologic disadvantage to any normal tissues remaining in the field when an entire dose is delivered in a single large fraction.
Postoperative Irradiation
Postoperative irradiation improves locoregional control and survival in several settings important to gynecologic oncologists. In vulvar cancer, postoperative pelvic and groin irradiation reduces the risk of groin recurrence and improves the survival rate of patients with multiple positive inguinal nodes (55). In endometrial cancer, postoperative pelvic irradiation reduces the incidence of pelvic recurrence in patients with high-risk disease (8,9,11). In cervical cancer, postoperative pelvic irradiation reduces the incidence of pelvic recurrence in patients with lymph node involvement and in those with high-risk features in the primary tumor (6,7).
Combination Approaches
Combined surgery and radiation therapy is optimized when the treatment plan exploits the complementary advantages of the two treatments. This requires close cooperation between specialists at the time of the patient’s initial evaluation. Because the morbidity of combined therapy is often greater than that of single-modality therapy, combined treatment should usually be limited to situations in which a combined approach is likely to improve survival, permit organ preservation, or significantly reduce the risk of local recurrence compared with the expected results from treatment with either modality alone (58).
Physical Principles
Ionizing Radiations Used in Therapy
Ionizing radiations lie on the high-energy portion of the electromagnetic spectrum and are characterized by their ability to excite, or ionize, atoms in an absorbing material. The decay of radioactive nuclei can produce several types of radiation, including uncharged gamma (γ) rays, negatively charged beta (β) rays (electrons), positively charged alpha (α) particles (helium ions), and neutrons. The resulting ionizing radiations are exploited therapeutically in brachytherapy treatments (using 226Ra, 137Cs, 186Ir, and other isotopes) or to produce teletherapy beams (e.g., 60Co). The average energy of the photons produced by the decay of radioactive cobalt is 1.2 million eV (MeV).
Most external beam therapy is delivered via linear accelerators that produce photon beams (x-rays) by bombarding a target such as tungsten with accelerated electrons. Varying the energy of the accelerated electrons produces therapeutic x-rays of different energies. X-rays and γ-rays are both composed of photons and differ only in that x-rays are produced by extranuclear forces and γ-rays are produced by intranuclear forces.
Interactions of Radiation with Matter
X-rays and γ-rays
Photons interact with matter by means of three distinct mechanisms: The photoelectric effect, Compton scatter, and pair production.
The photoelectric effect is most important at energies used for diagnostic purposes. Absorption by the photoelectric effect is proportional to Z3, where Z is the atomic number of the absorbing material. This effect is responsible for the increased absorption of bone that provides contrast between bone and soft tissue with diagnostic x-ray beams of 250 kV or less. However, the increased bone absorption, high skin dose, and poor penetration with such beams make them unsuitable for most modern therapeutic applications. Superficial kilovoltage radiation beams, delivered using a transvaginal cone, are occasionally used for patients with large bleeding exophytic tumors to achieve hemostasis before definitive treatment (59).
Modern therapeutic beams of 1 to 20 megavolts (MV) produce photons that interact with tissues primarily by Compton scatter. In this process, incident photons interact with loosely bound outer-shell electrons, ejecting them from the atom. Both the photon and the electron go on to interact with other atoms, causing additional ionizations. Compton-scatter absorption is independent of Z, but varies according to the density of the absorbing material. This accounts for the poor contrast of radiation portal verification films.
Photons that are absorbed by Compton scatter produce an increasing number of scattered electrons and ionizations as they penetrate beneath the surface of an absorbing material. This creates a buildup region just below the surface that is responsible for the skin-sparing characteristic of modern high-energy therapy beams (Fig. 4.6). The maximum dose from a megavoltage beam is reached at 0.5 to 3 cm below the skin surface, depending on the photon energy. At greater depths, the dose decreases at a fairly constant rate that is related to the beam energy. The greater skin-sparing effects and penetration of beams with energies of 15 MV or greater make such beams particularly useful for pelvic treatment.
Pair production absorption is related to Z2. In soft tissue, this type of absorption begins to dominate only at photon energies of more than approximately 30 MeV, so pair production is of limited importance in the current radiation therapy planning.
Figure 4.6 Depth dose curves for selected x-ray and γ-ray beams (top). As the energy increases, the depth of maximum dose (Dmax or D100) increases. For kilovoltage beams, the dose is maximum at the skin surface. With appositionally directed megavoltage beams (e.g., 60Co or 25-MeV photon beams), the maximum dose is reached at a depth beyond the skin surface, producing skin sparing. High-energy beams also penetrate more deeply, making them more useful for treatment of deep-seated pelvic tumors. Depth dose curves for electron beam fields of selected energies (bottom). The depth of maximum dose increases with increasing energy. At depths just below the maximum, the dose falls off rapidly, sparing deeper tissues.
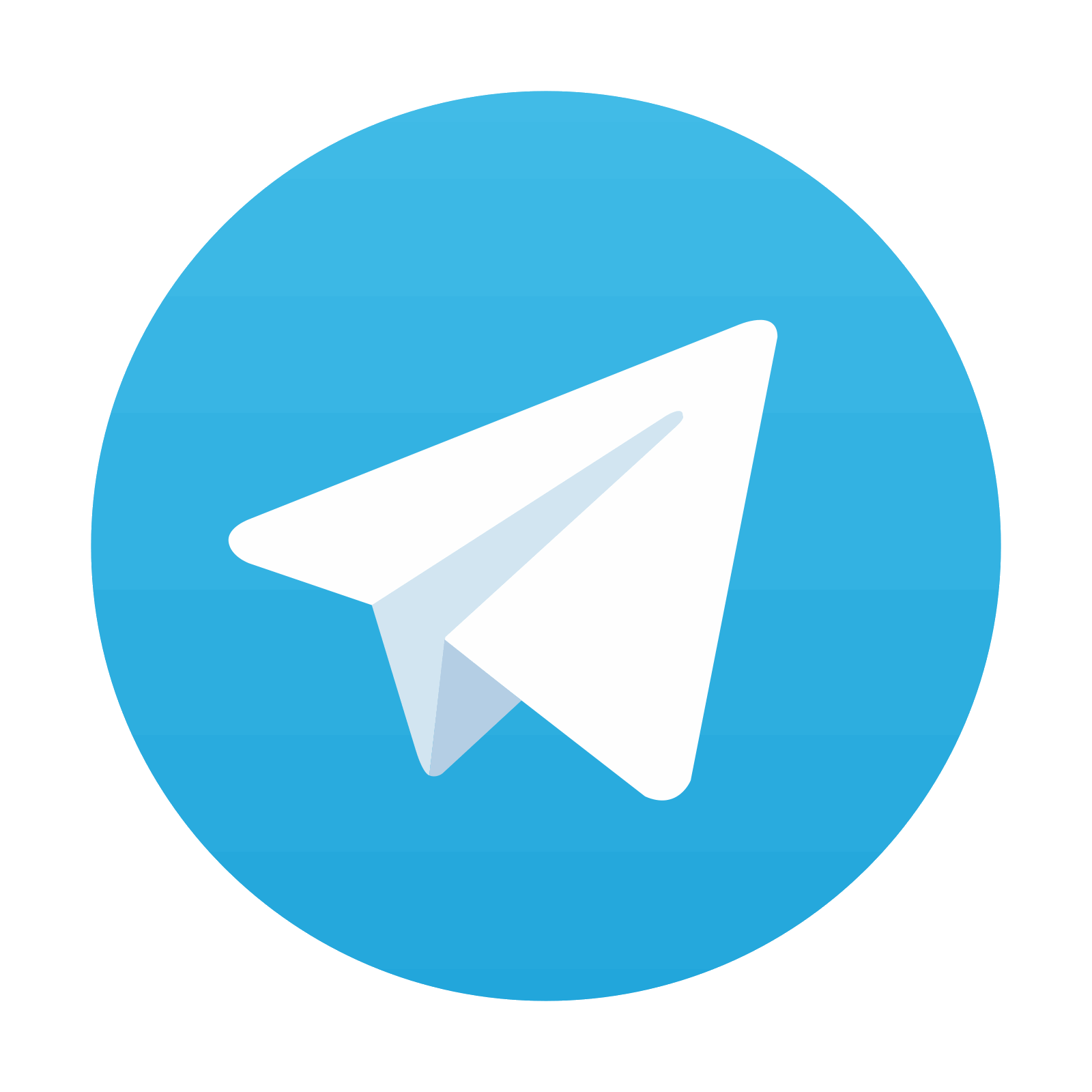
Stay updated, free articles. Join our Telegram channel
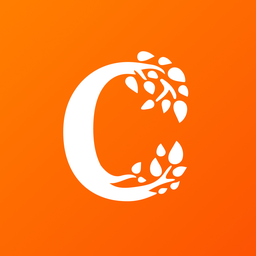
Full access? Get Clinical Tree
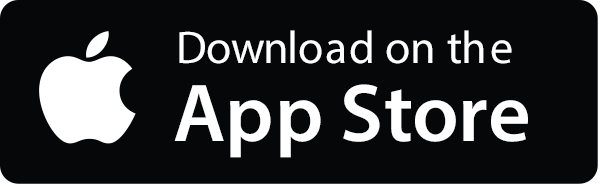
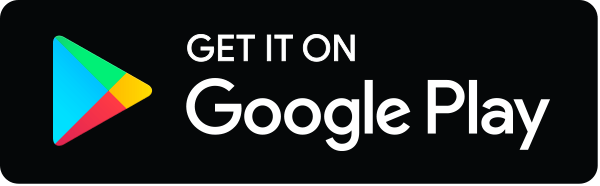