Pathogenesis of Diabetic Neuropathy
Phillip A. Low
The diabetic neuropathies are those associated with the diabetic state. Although the neuropathies are clearly related to the presence, duration, and severity of hyperglycemia, controversies exist on the types of diabetic neuropathies and their pathogenesis. Diabetic neuropathies have many phenotypes (Table 50.1). Distal sensory neuropathy is the most common variety of neuropathy, with mild distal sensory impairment and minimal motor deficits. This category comprises greater than 50% of all diabetic neuropathies. Distal small fiber neuropathy is also common; it is characterized paradoxically by a combination of distal positive symptoms, including painfulness and impairment in both pain and temperature perception. Painfulness occurs in many types of diabetic neuropathies. The asymmetric neuropathies are distinctive and are likely due to a combination of microvascular disease, compression injury, and immune-mediated mechanisms. Despite the profusion of manifestations and types of neuropathy, the common garden-variety distal sensory neuropathy is present in essentially all varieties of diabetic neuropathies and comprises the major focus of this chapter.
TABLE 50.1. Types of Diabetic Neuropathies | ||||
---|---|---|---|---|
|
UNIQUE CHARACTERISTICS OF THE PERIPHERAL NERVE
Because the peripheral nerve axons are extremely long relative to their diameters and are a great distance from their parent cell bodies (in dorsal root ganglion, autonomic ganglion, and motor neurons), their component motor, sensory, and autonomic axons, especially distally, are exquisitely dependent on endoneurial microenvironment for their blood supply, oxygenation, nutrition, and the removal of toxic metabolic products. The peripheral nerve is physiologically unique in several ways, information that has an important bearing on its response as a target to chronic hyperglycemia.
Resistance to Ischemic Fiber Degeneration
The nerve is supplied by a dual system of microvessels. It has an intrinsic interconnecting system, connected via arterioles and venules to an epineurial system, the extrinsic system (1). The dual system, low energy requirements, and relatively large energy stores together confer on the nerve a large safety factor in its resistance to ischemic fiber degeneration. Earlier experimental data in which peripheral nerve was undercut or the regional supply was ligated demonstrated that nerve fiber degeneration did not occur even when long stretches of nerve were deprived of their intrinsic supply either by arterial ligation (2) or by extensive nerve trunk mobilization with undercutting of connecting blood supply (3).
Lack of Autoregulation
Autoregulation refers to the maintenance of constant blood flow, in spite of changes in blood pressure [within a blood pressure
range (the autoregulated range)]. In most tissues, autoregulation is achieved by dynamically varying arteriolar tone and by myogenic rather than neurogenic mechanisms. There is a characteristic blood flow:blood pressure curve, which is flat within the autoregulated range and sloping at either end. We demonstrated a curvilinear relationship of nerve blood flow to blood pressure, a relationship that is explainable on the basis of a passive nonautoregulating system (4). This finding has subsequently been confirmed in anesthetized (5,6) and nonanesthetized rats (7).
range (the autoregulated range)]. In most tissues, autoregulation is achieved by dynamically varying arteriolar tone and by myogenic rather than neurogenic mechanisms. There is a characteristic blood flow:blood pressure curve, which is flat within the autoregulated range and sloping at either end. We demonstrated a curvilinear relationship of nerve blood flow to blood pressure, a relationship that is explainable on the basis of a passive nonautoregulating system (4). This finding has subsequently been confirmed in anesthetized (5,6) and nonanesthetized rats (7).
Nerve Is a Nutritive-Capacitance Vascular Bed
Another unique characteristic of nerve microvasculature is its nutritive capacitance system (5). This is a disadvantaged system where a small change in blood volume results in a disproportionate change in nerve blood flow. The morphologic basis of this system appears to be the large-diameter capillary whose diameter exceeds that of the erythrocyte. The median diameter of the endoneurial capillary is 8 to 9 μm (8), considerably larger than that of erythrocytes (about 7 μm). In other tissues the capillary is smaller than the erythrocytes, resulting in a squeezing action that enhances oxygen release. The large diameter with poorly developed arteriolar smooth muscle (9) results in inefficient oxygen release.
Nerve Can Be Sustained on Anaerobic Metabolism
The peripheral nerve is metabolically unique, being able to function relatively well on anaerobic metabolism and having powerful adaptive mechanisms. Compared with rat brain, the nerve has about 10% of the brain’s oxygen requirements but similar energy stores (10,11). When maximally active, the nerve increases its energy demands less than 100%, whereas the brain increases its demands severalfold (11). The relatively large energy stores and the low resting and maximal energy expenditure enable the nerve to function quite well on anaerobically generated high-energy phosphate. It is likely that the low-density capacitance system developed because of the low metabolism of the nerve. We have demonstrated that the nerve will conduct impulses for many additional minutes when energy substrates are increased, as in diabetes (11).
UNIQUE CHARACTERISTICS OF HUMAN DIABETIC NEUROPATHY
There are a number of unique characteristics of human and experimental diabetic neuropathy. Four of these are relevant to the pathogenesis of diabetic neuropathy:
Susceptibility to entrapment/compression neuropathies
Susceptibility to ischemic fiber degeneration
Insulin-induced neuritis
Resistance to Ischemic Conduction Failure
When the limb is rendered ischemic, paresthesias develop followed by the loss of sensory then motor functions. The electrophysiologic correlate is a failure of nerve conduction. Both diabetic humans and animals manifest resistance to ischemic conduction failure (RICF) (11,14). Nerve impulses continue to conduct for minutes in diabetic individuals after those in normal individuals have failed to do so. The clinical correlate is that patients are more resistant to paresthesias and retain nerve function for minutes beyond normal subjects.
MECHANISM
The mechanism of RICF likely relates to the unique metabolic characteristics described previously. Diabetic nerves have a severalfold increase in nerve energy substrates (glucose, fructose, glycogen) (11). Their energy requirements are sufficiently low to permit them to continue to conduct impulses on adenosine triphosphate (ATP) derived from anaerobic metabolism (1). Their energy requirements may also be downregulated. The relatively large endoneurial space (relative to brain) results in less susceptibility to lactate- and potassium-induced conduction failure (11).
Susceptibility to Entrapment/Compression Neuropathies
Diabetic nerve trunks are unduly susceptible to focal neuropathies that occur in a limited number of circumstances (15). Focal neuropathies occur due to repetitive compression, as occurs with leaning on the ulnar nerve at its groove and pressure of the peroneal nerve against the fibular neck (15). Entrapment can occur within the carpal tunnel as with the median nerve, causing carpal tunnel syndrome (15). A third category is the acute onset of focal noncompressive neuropathy, e.g., third cranial nerve.
MECHANISM
The precise pathophysiology is not known. Circumstantial evidence suggests that the combination of ischemia and mechanical susceptibility might be responsible. Evidence for microvascular ischemia clinically includes the acute or subacute onset of, for example, third-nerve palsy in vasculopathic older patients. Pathologic evidence is limited but includes the presence of microinfarcts, multifocal pathology, and fibrinoid change (16). Such changes have been described in two diabetic patients; one died shortly after the onset of oculomotor palsy (17); in the second, a 73-year-old man with diabetes who died shortly after the onset of a focal limb neuropathy, the obturator, femoral, sciatic, and posterior tibial nerves were examined (18). Combined changes of florid round cell infiltration and microvascular changes have been interpreted as being due to a combination of microvascular ischemia and immune-mediated mechanisms. Three sets of findings are consistently found in chronic diabetic neuropathy: first, a thickening of basal lamina (19); second,
endothelial-cell and smooth-muscle proliferation (20,21) with increased variance of capillary caliber and the closing of some capillaries (20); third, the tendency of fiber loss to be multifocal rather than diffuse (22,23). The combination of microvascular ischemia, connective tissue changes (24), and myelin alterations could presumably render the nerve trunk susceptible to compression injury.
endothelial-cell and smooth-muscle proliferation (20,21) with increased variance of capillary caliber and the closing of some capillaries (20); third, the tendency of fiber loss to be multifocal rather than diffuse (22,23). The combination of microvascular ischemia, connective tissue changes (24), and myelin alterations could presumably render the nerve trunk susceptible to compression injury.
Excessive Susceptibility to Ischemic Fiber Degeneration of Diabetic Nerves
Ischemia of mild-to-moderate severity is associated with excessive susceptibility to ischemic fiber degeneration. The latter is reported to occur in animals with chronic hyperglycemia in excess of 16 weeks but not with hyperglycemia of shorter duration (25,26). Other studies report excessive susceptibility much earlier in the course of diabetes. Reports include excessive susceptibility to ischemic fiber degeneration in response to epineurial application of endothelin in rats with a duration of hyperglycemia of only 6 to 8 weeks (27), and with the in vitro study showing that a medium with increased glucose causes intracellular acidosis with delayed recovery (28).
The human equivalent of susceptibility to ischemic fiber degeneration is not well documented.
Insulin-Induced Neuropathy
Infrequently, an acute painful neuropathy develops within 1 month of the institution of tight glycemic control with insulin therapy in a diabetic patient who previously did not have a neuropathy (29,30,31). The neuropathy is usually that of a painful distal sensory polyneuropathy, although it also can be a severe generalized neuropathy.
Recent experimental studies provide some insight into the mechanism of insulin neuritis. Insulin administration reproducibly causes acute endoneurial hypoxia by increasing nerve arteriovenous flow and reducing nutritive flow of normal nerves (32). The diabetic state confers resistance to this effect. Within 1 month of commencement of insulin treatment, this susceptibility to the endoneurial hypoxic effect of insulin recurs (32). Endoneurial hypoxia has been demonstrated in experimental and human diabetic neuropathy (33,34). We had suggested that diabetic nerve fibers survive under these hypoxic conditions because of the greatly increased energy substrate stores in experimental and human diabetes (11,35), coupled with the low energy requirements of peripheral nerve (1). Anaerobic metabolism suffices under these circumstances. Insulin treatment causes transient hypoxia in normal but not diabetic nerves (32). With continued insulin treatment, energy substrates are normalized and the susceptibility to insulin-induced endoneurial hypoxia returns in diabetic nerves. The hypoxic tissues with normal energy substrates fare poorly because anaerobic metabolism is inefficient (1), and there may be sufficient hypoxia to result in fiber degeneration.
PATHOGENESIS OF DIABETIC NEUROPATHY
The pathogenesis of the common, garden-variety distal sensory diabetic neuropathy is likely multifactorial. Hyperglycemia is central to any pathogenetic scheme. There are sufficient experimental and observational data to warrant consideration of the following mechanisms in mediating the effects of chronic hyperglycemia:
Genetic predisposition
Nerve hypoxia/ischemia
Oxidative stress
Overactivity of polyol pathway
Increased advanced glycation end products
Deficiency of γ-linolenic acid
Protein kinase C, especially increase in β-isoform
Growth factor(s) deficiency
Dysimmune mechanisms
Genetic Predisposition
One patient with lifelong severe hyperglycemia is free of neuropathy; another has florid neuropathy within a few years of onset of hyperglycemia; another patient with a strong family history of diabetes may actually develop neuropathy before diabetes is diagnosable. Although these types of observations suggest a genetic predisposition to the development of neuropathy, the available data are very limited. Most of the studies to date have focused on the genetics of inheritance of type 1 and type 2 diabetes.
Nerve Ischemia/Hypoxia
EXPERIMENTAL DIABETIC NEUROPATHY
Vasoregulation of nerve microvessels is regulated at the arteriolar, venular, and endothelial cell levels. In the diabetic state, endothelial changes include those of AGE-RAGE-NF-κB [advanced glycosylation end products (AGE)–AGE receptor- nuclear factor κB (NF-κB)], cell adhesion molecules, nitric oxide, and eicosanoids. Experimental diabetic neuropathy results in a reduction in nerve blood flow of about 50% (33,36), which begins by the first week of diabetes (36) and affects the cell body of sensory and sympathetic neurons as well as the axon (37). In diabetes, the combination of increased whole-blood or plasma viscosity, increased aggregability, and reduced red blood cell deformability results in a reduction of blood flow and stagnation hypoxia (1).
Regional nerve blood flow is regulated by systemic blood pressure (4) and the balance between neural vasoconstrictors and vasodilators. Vasoconstriction is known to be mediated by epineurial α-adrenergic (38,39), vasopressin (40), and endothelin receptors (41). The 50% effective concentration (EC50) of endothelin, using identical methodology, is about three orders of magnitude lower, indicating greater potency, than that of adrenoreceptors (41). The EC50 of vasopressin is of an order similar to that of endothelin. These potent vasoconstrictor actions are balanced by vasodilatation and are mediated by calcitonin gene-related peptide (42), substance P (42), nitric oxide (NO) (43), and the prostaglandins (44).
Alterations in microvessels in experimental diabetic neuropathy have been reported. These are reported to be excessively sensitive to α-adrenergic agonists (45) and endothelin (46,47). The nerve conduction and blood-flow deficits are prevented by specific antagonists to endothelin (48) and adrenoreceptors (49). Deficits in vasodilators are supported by restoration of blood flow and nerve conduction by an NO donor (50) and vasodilator prostaglandins (51). The reduction of NO is likely the most important in its magnitude and implications (43). The polyol pathway is overactive and competes with nitric oxide synthase for NADPH (reduced form of nicotinamide adenine dinucleotide phosphate). By siphoning off NADPH, chronic hyperglycemia results in a deficiency of NO in nerve endothelium (43,50). Endoneurial NO deficiency has a number of important consequences in the microvasculature. It normally inhibits the expression of P-selectin, intercellular adhesion molecule-1 (ICAM-1), vascular cell adhesion molecule-1 (VCAM-1), and other adhesion molecules (52), a mechanism that is mediated
through inhibition of protein kinase C and by preventing the activation of NF-κB (53). NO also inhibits the cytoassembly of NADPH oxidase (54), thereby attenuating the release of superoxide by leukocytes (55). Inhibition of NO, therefore, explains a number of changes seen in experimental diabetic neuropathy (expression of adhesion molecules, activation of NF-κB, expression of cytokines, and generation of superoxide anions).
through inhibition of protein kinase C and by preventing the activation of NF-κB (53). NO also inhibits the cytoassembly of NADPH oxidase (54), thereby attenuating the release of superoxide by leukocytes (55). Inhibition of NO, therefore, explains a number of changes seen in experimental diabetic neuropathy (expression of adhesion molecules, activation of NF-κB, expression of cytokines, and generation of superoxide anions).
HUMAN DIABETIC NEUROPATHY
Three main pathologic alterations affect human diabetic peripheral nerve. First, thickening of endoneurial capillary wall and thickening and reduplication of basal lamina have consistently been found (56,57), especially in more recent studies (16,58). Basal lamina thickening, although characteristic, is not specific to the diabetic state, occurring especially with aging and a number of other neuropathies. Second, alterations in capillaries are consistently present. These changes include a reduction in capillary density, reduced capillary area, and capillary closure (16,20,58). Third, the pattern of fiber loss is multifocal, suggestive of ischemic fiber loss (22,23). In a three-dimensional reconstruction of the distribution of fiber loss using autopsy specimens from lumbosacral trunk to sural nerve biopsy specimens, the changes seen in sural nerve manifest the cumulative loss of fibers, whereas multifocal fiber loss is better appreciated in more proximal nerve trunks (59). Although these alterations have been well defined, their specificity has been questioned (60). These studies are all important in attempting to define morphometric abnormalities of endoneurial vessels and fibers. They suffer from their ability to define the results but inability to define the pathogenetic process.
The most concerted and coherent studies of perfusion and oxygenation of human diabetic nerves have been undertaken by the Sheffield group. They were the first to demonstrate reduced oxygen tension in human diabetic neuropathy. They measured endoneurial oxygen tension in exposed sural nerve of 11 patients with diabetic neuropathy (34). Correlative pathologic studies in a separate study were supportive, showing reduced endoneurial capillary density and changes in capillary basement membrane and endothelial cells and in the derived total diffusion barrier that was significantly impaired in the nerves of patients with severe diabetic neuropathy as compared with control subjects (58). Blood flow and oxygen tension recordings in the operating room are not trivial. Recordings using laser Doppler methodology have been reported to show no change or even a slight increase in blood flow and oxygen tension (61). These results are un-interpretable in diabetic neuropathy, because it measures total and not nutritive flow, where shunt flow may actually be increased (62).
A number of innovative approaches have been developed to measure flow and oxygenation. Nerve photography and fluorescein angiography demonstrate delayed fluorescein appearance time and intensity of fluorescence in human diabetic neuropathy (62). These investigators observed direct epineurial arteriovenous shunting in the majority of diabetic neuropathic patients. A novel approach using microlightguide spectrophotometry to measure intravascular oxygen saturation and blood flow in human sural nerve after an intravenous injection of sodium fluorescein has confirmed impaired nerve blood flow and tissue deoxygenation. There was a correlation between rise time, nerve oxygen saturation, glycemic control, and sural nerve sensory conduction velocity (63).
THERAPEUTIC APPLICATIONS
Angiotensin-converting enzyme (ACE) inhibition with lisinopril administered to 13 hypertensive patients with diabetic neuropathy was reported to improve nerve conduction indices and sensory perception thresholds (64). A small, randomized, double-blind, placebo-controlled trial on the effect of the ACE inhibitor trandolapril on 41 normotensive patients with type 1 or type 2 diabetes and mild neuropathy has appeared (65). Changes in the neuropathy symptom and deficit scores, vibration-perception threshold, peripheral-nerve electrophysiology, and cardiovascular autonomic function were assessed at 6 and 12 months. The primary end point was the change in peroneal nerve motor conduction velocity. Peroneal motor nerve conduction velocity (p = 0.03) and M-wave amplitude (p = 0.03) increased, the F-wave latency (p = 0.03) decreased, and sural nerve action potential amplitude increased (p = 0.04) significantly after 12 months of treatment with trandolapril as compared with response to placebo.
The study is interesting and has potentially important implications. There is controversy over whether ischemia is a central mechanism or is one of many mechanisms in the production of diabetic neuropathy. In view of the strength of the evidence of the benefits of ACE inhibition on renal function, it might be difficult to set up a specific study on neuropathy, in contrast to a neuropathy piggyback study similar to the Diabetes Control and Complications Trial.
Oxidative Stress
There is considerable evidence of oxidative stress in both experimental and human diabetic neuropathy. We will summarize the evidence in non-neural tissues followed by data on neural tissue. The data on non-neural tissues come from studies of plasma; erythrocytes; and primarily heart, liver, kidney, and pancreas. These studies have focused on evidence of increased lipid peroxidation and alteration in the patterns of glutathione and antioxidant enzymes. Evidence of oxidative stress may be manifested as a reduction or an increase in antioxidant enzymes (66). Not all experts espouse the role of oxidative stress. One view is that the predominant stress is reductive, characterized by an increase in the NADH/NAD+ (reduced form of nicotinamide adenine dinucleotide oxidized form of nicotinamide adenine dinucleotide) ratio (67). Another view, proposed by John Baynes (68), is thoughtful and complicated. He recognizes that increases in glycoxidation and lipoxidation products suggest that oxidative stress is increased in diabetes but that the data are best explained as an increase in carbonyl stress.
OXIDANT STRESS IN HUMAN DIABETES
Oxidative stress refers to a shift in the pro-oxidant:antioxidant status toward the former. Plasma levels of lipid peroxide are increased in human diabetes (69,70,71,72,73,74) and occur early, even in young persons with diabetes (75). The highest levels were reported in patients with microvascular angiopathy, as manifested as retinopathy or microalbuminuria; lowest in those patients with diabetes without angiopathy (69,76); and normal in patients with well-controlled diabetes (69). The relationship may relate to the observation that low-density lipoproteins of patients with diabetes are significantly more oxidizable than are those of controls, an abnormality that is correctable by 6 weeks of treatment with the antioxidant probucol (77). Presumably, patients with diabetes with angiopathy who have higher levels of low-density lipoproteins will have the greatest lipid peroxidation.
More recent data are more consistent with increased oxidant stress being secondary to metabolic status rather than to complications (78), including a positive correlation with glycosylated hemoglobin and a negative correlation of malondialdehyde with glutathione peroxidase (79). Recent work has focused on combining measurements of lipid peroxidation (increased)
with indices of total antioxidant defense (reduced) in diabetes (80,81). Additional emphasis has been placed on evaluation of indices that reflect specific mechanisms and tissues affected by oxidant stress. Measurements include those of increased activation of the oxidative stress-sensitive transcription factor NF-κB (82). Greater importance also is placed on products that specifically reflect neural or DNA damage, such as 4-hydroxynonenal and 8-oxo, 2′-deoxyguanosine in relevant tissue (83). Urinary 8-oxo, 2′-deoxyguanosine excretion and the 8-oxo, 2′-deoxyguanosine content in the mononuclear cells from diabetic patients with complications were higher than those from diabetic patients without complications (84,85).
with indices of total antioxidant defense (reduced) in diabetes (80,81). Additional emphasis has been placed on evaluation of indices that reflect specific mechanisms and tissues affected by oxidant stress. Measurements include those of increased activation of the oxidative stress-sensitive transcription factor NF-κB (82). Greater importance also is placed on products that specifically reflect neural or DNA damage, such as 4-hydroxynonenal and 8-oxo, 2′-deoxyguanosine in relevant tissue (83). Urinary 8-oxo, 2′-deoxyguanosine excretion and the 8-oxo, 2′-deoxyguanosine content in the mononuclear cells from diabetic patients with complications were higher than those from diabetic patients without complications (84,85).
Glutathione (GSH) is reduced in erythrocytes from patients with type 2 diabetes, with a corresponding increase in oxidized glutathione (GSSG) (86,87). In subsequent studies, this same group additionally demonstrated reductions in the glutathione synthesizing enzyme, γ-glutamylcysteine synthetase, and the transport of thiol [S-(s,4-dintrophenyl)glutathione] in erythrocytes of these patients. These abnormalities were reversible with improved glycemic control. They also demonstrated that a medium high in glucose augments the toxicity of xenobiotics on K562 cells, associated with reduction in both the enzyme and its mRNA (88). Lipid peroxidation is increased and antioxidant enzymes are reduced in the erythrocytes of persons with type 2 diabetes. Changes in GSH and antioxidant enzymes occur early, even at onset of type 1 diabetes (75).
Erythrocyte cuprozinc superoxide dismutase (SOD) is reduced in patients with type 2 diabetes (70,89), and catalase is variably reduced (89). It is suggested that this reduction is mediated by the accumulation of intracellular H2O2 (90). Changes in SOD occur early (75).
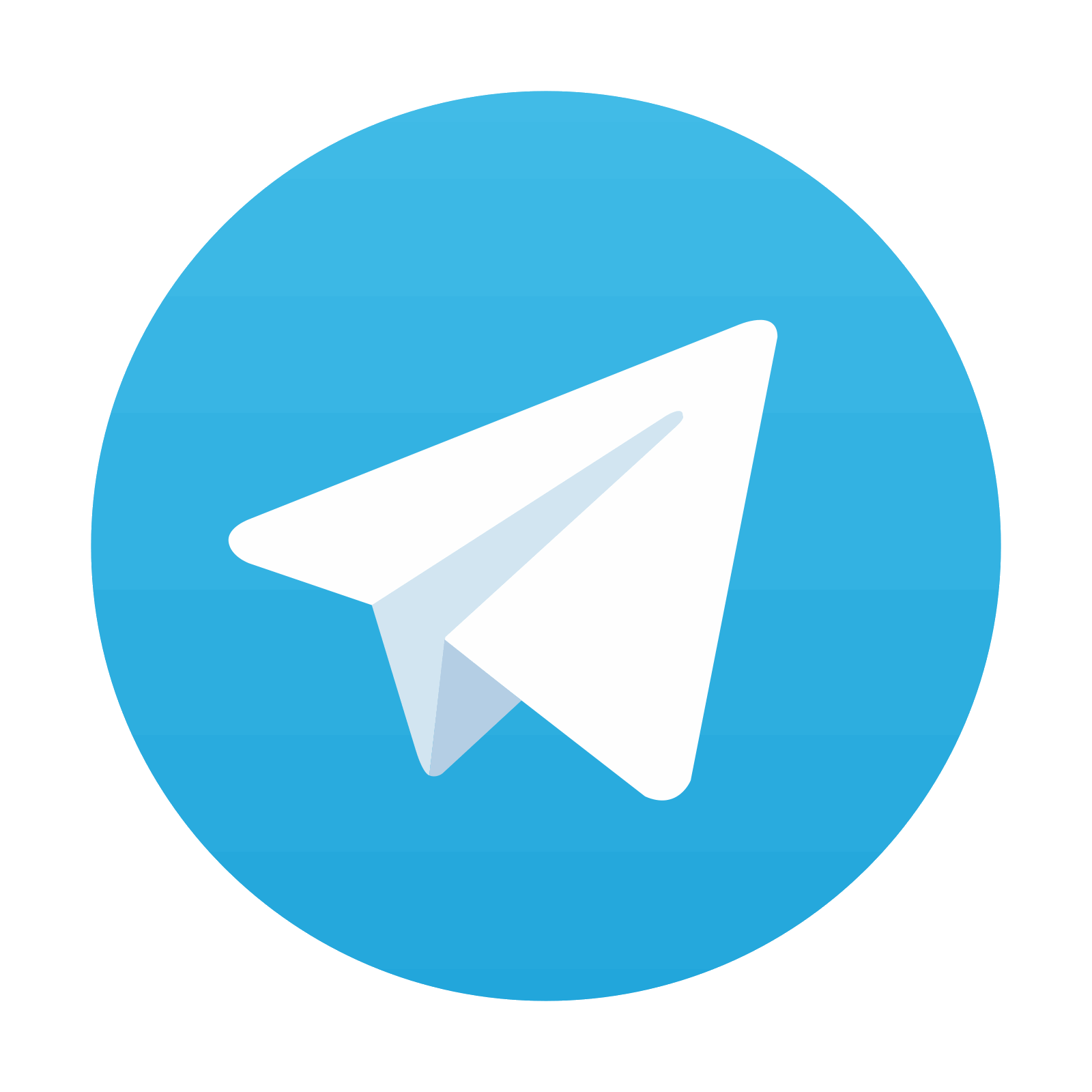
Stay updated, free articles. Join our Telegram channel
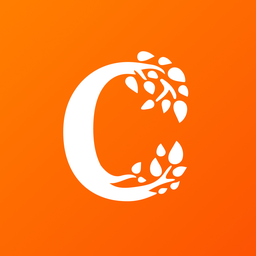
Full access? Get Clinical Tree
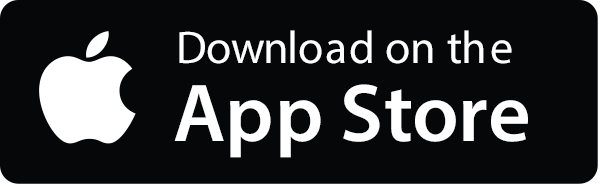
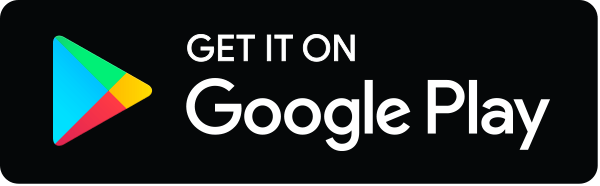
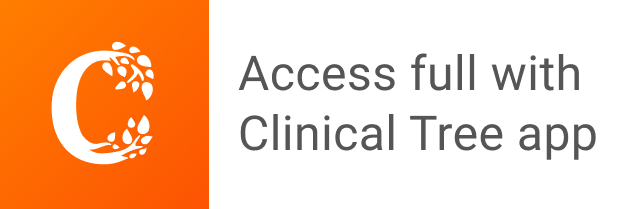