Biology of Skeletal Muscle
Alison C. Jozsi
Laurie J. Goodyear
ANATOMY
The human body contains more than 600 different skeletal muscles, collectively comprising the largest single organ of the body. The morphology of skeletal muscle at both the microscopic and macroscopic levels is intimately tied to its primary function, which is contractile activity. Each skeletal muscle in the body is covered by a dense connective tissue layer called the epimysium (Fig. 14.1). Extending inward from the epimys-ium is the perimysium, which surrounds small bundles of individual muscle fibers. Each individual muscle fiber within these bundles is surrounded by the endomysium, a thin layer of connective tissue. Within the endomysium is the cell membrane, which in muscle is called the sarcolemma. A dense capillary network extends throughout skeletal muscle, with several capillaries surrounding each muscle fiber. Individual cylindrical fibers do not always extend from one end of the muscle to the other; therefore, the connective tissue surrounding the muscle fiber bundles may be important for translating the mechanical forces of contraction throughout the length of the entire muscle group (1).
Muscle fibers contain dense networks of contractile proteins that are arranged precisely to achieve muscle contraction and body movement. Each muscle fiber is a single muscle cell. Fibers are roughly cylindrical, with the diameter of the fiber ranging between 10 and 100 μm (1). The length of fibers is variable, with some fibers extending the entire length of the muscle and to a length of 35 cm. The strength of a muscle fiber is directly proportional to its cross-sectional area, which can change dynamically commensurate with neuromuscular activity and muscle use.
Skeletal Muscle Organelles
Some of the organelles in the muscle fiber are similar to those in other eukaryotic cells but are named differently, such as the sarcolemma, the sarcoplasm, and the sarcoplasmic reticulum, which correspond to the plasma membrane, the cytoplasm, and the endoplasmic reticulum, respectively, of other eukaryotic cells. The sarcoplasm of the muscle fiber differs from the cytoplasm of most eukaryotic cells in that it contains a large quantity of stored glycogen. The sarcoplasmic reticulum is a longitudinal network of tubules within the muscle fiber that runs parallel to and surrounds the myofibrils (Fig. 14.2). The sarcoplasmic reticulum stores calcium, which is released during excitation of the muscle fiber. The transverse tubule system (T tubule) is a tubular network of membranous sacs that intertwines the myofibrils and facilitates the transmission of nerve impulses, as well as the transport of extracellular glucose, oxygen, and other electrolytes, to the individual myofibrils. The T tubules are formed by invaginations of the sarcolemma into and around the muscle fibers (1).
Although many of the organelles inside muscle fibers are common to most eukaryotic cells, muscle fibers are unique in many ways. For example, unlike most eukaryotic cells, which contain single, centrally located nuclei, muscle fibers are multinucleated, and these nuclei are located peripherally along the cell (Fig. 14.2). The nuclei likely act as local governing centers of cellular functions and adaptation to various stimuli and also function as integrated centers that communicate stimuli along the length of the muscle fiber to enable cohesive adaptation (2). The ratio of nuclei to cytoplasmic domain in a muscle fiber seems to be tightly regulated and dependent on the neuromuscular
activity of the muscle fiber (2). Although mature muscle cells are postmitotic, skeletal muscle tissue has unique regenerative capacity afforded by quiescent myogenic cells called satellite cells that lie between the sarcolemma and the basal lamina of muscle fibers. The precise chemical and physical signals that activate satellite cells from their quiescent state to proliferate and differentiate into mature muscle fibers are still unclear.
activity of the muscle fiber (2). Although mature muscle cells are postmitotic, skeletal muscle tissue has unique regenerative capacity afforded by quiescent myogenic cells called satellite cells that lie between the sarcolemma and the basal lamina of muscle fibers. The precise chemical and physical signals that activate satellite cells from their quiescent state to proliferate and differentiate into mature muscle fibers are still unclear.
Neuromuscular Junction
Muscle contraction occurs following the initiation and propagation of an action potential along a motor nerve to its endings on muscle fibers. The motor nerve enters the muscle and branches out between the muscle fascicles; these branches may then innervate a single muscle fiber or hundreds of muscle fibers. A motor unit is composed of a single nerve fiber and the cluster of muscle fibers that the nerve innervates. The site of neural innervation at the muscle cell surface is called the neuromuscular junction or the motor end plate (Fig. 14.3). The nerve axon terminus contains many mitochondria and synaptic vesicles containing the neurotransmitter acetylcholine. The space between the nerve axon terminus and the muscle fiber is called the synaptic cleft. To increase the surface area for acetylcholine receptors in the synaptic cleft, the sarcolemma is extensively folded in the region of the neuromuscular junction. There is a marked density of nuclei, mitochondria, ribosomes, and glycogen molecules in the sarcoplasm below the synaptic cleft (3).
Sarcomeric Structure
Each muscle fiber is composed of thousands of myofibrils, each of which is a cylindrical filament that runs parallel to the longitudinal axis of the muscle fiber (Fig. 14.4A). The myofibrils consist of a specialized arrangement of myosin and actin filaments, also called thick and thin filaments, respectively. These filaments interact to produce muscle contraction. Each actin filament is connected at one end to a protein structure called the Z line (or Z disc). The area containing actin and myosin filaments between two Z lines is called the sarcomere. Sarcomeres exist as repeating units along the full length of the myofibril and represent the functional (contraction-producing) unit of the muscle (4). The Z line is composed of two major proteins, α-actinin and desmin, which hold adjacent sarcomeres together. Titin is another very important myofibrillar protein that provides a lattice-like support structure that enables the side-by-side arrangement of myosin and actin. The actin filaments extend outward from the Z line into the sarcomere, and myosin filaments surround the actin filaments. The area on either side of the Z line
that is occupied only by actin filaments, with no overlap by myosin filaments, is the I band (“I” for isotropic; does not alter polarized light). The A band is the dark area in the middle of a sarcomere where the actin filaments are overlapped by myosin filaments (“A” for anisotropic; birefringent in polarized light) (1). When the muscle is not contracting, there is an area in the middle of the sarcomere occupied only by myosin filaments (H zone). When the muscle sarcomeres shorten, the actin filaments are pulled inward to completely overlap myosin filaments, and the H band disappears (4). The spatial arrangement of the thick myosin and thin actin filaments relative to one another, along with the other myofibrillar proteins that facilitate their interaction, yields a striated pattern observable under the light microscope (Fig. 14.4B).
that is occupied only by actin filaments, with no overlap by myosin filaments, is the I band (“I” for isotropic; does not alter polarized light). The A band is the dark area in the middle of a sarcomere where the actin filaments are overlapped by myosin filaments (“A” for anisotropic; birefringent in polarized light) (1). When the muscle is not contracting, there is an area in the middle of the sarcomere occupied only by myosin filaments (H zone). When the muscle sarcomeres shorten, the actin filaments are pulled inward to completely overlap myosin filaments, and the H band disappears (4). The spatial arrangement of the thick myosin and thin actin filaments relative to one another, along with the other myofibrillar proteins that facilitate their interaction, yields a striated pattern observable under the light microscope (Fig. 14.4B).
A myosin filament consists of approximately 200 myosin molecules lined up end to end and side by side. Myosin has been studied extensively, and it is the diverse features of this protein that lead to the tremendous variation in contractile velocity and force production with different fiber types. Each myosin molecule is composed of two identical heavy chains and four light chains (Fig. 14.5A). The heavy chains are cylindrical proteins twisted around each other in a double-helical formation, each with a globular head at one end. The globular head portion of the myosin protein is able to bind both actin and adenosine triphosphate (ATP), as well as to hydrolyze ATP via intrinsic adenosine triphosphatase (ATPase) activity (5). Two myosin light chains are associated with each globular head region of the heavy chains and are thought to regulate the ATPase activity of the myosin head, thereby influencing the speed of muscle contractions. The three-dimensional struc-ture of the myosin molecule is such that a portion of the cylindrical chain and the globular polypeptide head of the myosin molecule extend sideways from the helical body of the filament and form a cross-bridge to the actin filaments. Each cross-bridge is flexible at the place where the cylindrical arm begins to extend outward from the straight chain, as well as where the globular head meets this arm. These hinged regions enable both the extension of the globular myosin head away from the helical portion and movement of the myosin head when it is associated with the actin molecule, as is the case during contraction. The bundles of myosin filaments are twisted around each other so that each pair of myosin heads are separated by precisely 120 degrees, thereby ensuring that cross-bridges extend in all directions from the myosin filaments to interact with actin molecules (5).
The actin filament is composed of a double-helical arrangement of spherical actin monomers, each approximately 40 kilodaltons (kDa) (Fig. 14.5B). The actin monomers are called G-actin, and polymerized G-actin forms filamentous F-actin. Each G-actin monomer has one adenosine diphosphate (ADP) molecule bound to it; it is thought that this ADP molecule provides the binding site for the myosin cross-bridge. These active sites
are also staggered for optimal interaction with the axially separated myosin cross-bridges. Two other proteins, tropomyosin and troponin, are associated with the actin filament. Troponin molecules bind approximately every seventh actin monomer along the filament. Troponin is composed of three subunits, Tn-I, Tn-C, and Tn-T (1). The Tn-T subunit binds troponin to tropomyosin and thereby attaches tropomyosin to the actin polymer. The troponin-tropomyosin complex blocks the active sites on actin for myosin cross-bridge formation when calcium is not present. The Tn-C subunit of troponin binds calcium during excitation of the muscle, which results in a conformational change that frees the active site and allows contraction to occur. The Tn-I subunit can inhibit tropomyosin when calcium is not present.
are also staggered for optimal interaction with the axially separated myosin cross-bridges. Two other proteins, tropomyosin and troponin, are associated with the actin filament. Troponin molecules bind approximately every seventh actin monomer along the filament. Troponin is composed of three subunits, Tn-I, Tn-C, and Tn-T (1). The Tn-T subunit binds troponin to tropomyosin and thereby attaches tropomyosin to the actin polymer. The troponin-tropomyosin complex blocks the active sites on actin for myosin cross-bridge formation when calcium is not present. The Tn-C subunit of troponin binds calcium during excitation of the muscle, which results in a conformational change that frees the active site and allows contraction to occur. The Tn-I subunit can inhibit tropomyosin when calcium is not present.
CONTRACTION OF SKELETAL MUSCLE
Skeletal muscle fibers shorten or contract when calcium is released into the sarcoplasm. Calcium release is coupled to depolarization of sarcolemmal and transverse tubule membranes. Taken together, this series of events has been defined as “excitation-contraction coupling.” Membrane excitation occurs when the action potential reaches the nerve terminus, causing the synaptic vesicles in the nerve terminus to release acetylcholine into the synaptic cleft. The acetylcholine binds to acetylcholine-gated channels on the sarcolemma and increases the sarcolemmal permeability to sodium, resulting in membrane depolarization and the development of another action potential. The action potential and membrane depolarization is propagated across the entire sarcolemma and into the muscle fiber via the transverse tubule system and the sarcoplasmic reticulum (3). Translation of the action potential and subsequent depolarization of the sarcoplasmic reticulum culminates in the release of calcium into the sarcoplasm and the initiation of contraction.
Following release from the sarcoplasmic reticulum, the calcium ions bind the actin regulatory troponin-tropomyosin complex, thereby releasing the actin active sites so that actin-myosin cross-bridges may form. A basic description of mus-cle shortening or contraction can be provided by breaking the process into several steps (Fig. 14.6). The myosin ATPase enzyme in the globular myosin head cleaves a molecule of ATP to ADP + Pi (inorganic phosphate), the latter of which remains bound to the myosin head. The energy yielded from ATP hydrolysis causes a conformational change in the myosin head, during which the head extends perpendicular toward and attaches to the actin filament. When the myosin head attaches to the actin filament, the head moves inward toward the cylindrical arm of the myosin filament and pulls the actin filament in the same direction. The inward motion of the myosin head causes a conformational change that releases the bound ADP molecule, and a new ATP molecule binds. The binding of the new ATP molecule causes another structural change in the globular myosin head that releases the head from the actin active site. This cycle of ATP cleavage, myosin-actin attachment, and pulling actin forward repeats until the Z lines (to which the end of the actin filaments are attached) within each sarcomere have been pulled all the way in to the ends of the myosin filaments. This repeating process results in muscle contraction (5). With the cessation of the action potential, calcium is actively transported back into the sarcoplasmic reticulum, troponin can once again inhibit the actin-myosin binding site, and muscle relaxation begins.
When an action potential is propagated to all the nerve endings in a motor unit, all of the muscle fibers in the motor unit contract together. Therefore, motor units are modeled according to the intensity of the contraction required and the degree of motor control needed for various movements. In regions of the body where fine motor control is necessary, each nerve axon may innervate only one muscle fiber. Where coarser control is satisfactory but the development of great tension is desirable, as in moving a limb, larger motor units predominate, in which one motor nerve innervates clusters of muscle fibers (3).
TYPES OF SKELETAL MUSCLE FIBERS
The muscle fibers differ tremendously morphologically, biochemically, and physiologically. Different muscle fiber types can be characterized by histologic methods according to myofibrillar ATPase activity or aerobic or anaerobic enzyme activity. For example, a reciprocal staining pattern occurs with acid or alkaline preincubation of muscle cross-sections, followed by myosin ATPase staining that reflects the spectrum of fiber types in the muscle. By this method, skeletal muscle was first divided into two major fiber types, type I and type II. Type I fibers are usually red, and type II fibers are white, proportional to the myoglobin content in the muscle. These fiber types are, as stated above, reciprocal in their metabolic enzymatic activities; slow-twitch type I fibers have low myosin ATPase activity and high aerobic oxidative enzyme activity, whereas fast-twitch type II fibers have high ATPase activity, low oxidative enzyme activity, and high anaerobic glycolytic enzyme activity. Investigations over the past 40 years have demonstrated that classifying fiber types by myosin ATPase is an oversimplification, and, in fact, muscle fibers commonly express several myosin isoforms at one time. Muscle fibers display a continuum of myosin isoforms, and a given fiber can alter its myosin ATPase, metabolic, and physiologic characteristics along either direction of this continuum, related to the stimuli received. For example, the human soleus muscle is a postural muscle and as such, contains primarily slow-twitch, oxidative type I fibers. However, under non-weight-bearing conditions, the soleus begins to express type IIa fibers, an intermediate fiber type that tends to be high in oxidative and glycolytic enzymes and in ATPase activity. With time, the soleus will progress further to a type IIb fiber phenotype, acquiring even more glycolytic and fast-contractile characteristics.
Skeletal muscle fibers display tremendous morphologic and biochemical plasticity in response to different stimuli, including altered energy status, gravity/mechanical load, neural stimulation, and intracellular calcium. It is clear that motor nerve activity influences muscle growth and fiber type by regulating
muscle gene expression (6). The regulation of fiber type-specific gene expression likely occurs through both the pattern of electrical activity (and the resultant calcium release) and the trophic factors released at nerve termini. For example, it has been shown that reinnervation of muscles by motor neurons with a different firing pattern results in muscle fiber transformation. Similarly, remodeling of muscle fiber type distribution, myofi-brillar proteins, mitochondria number, and metabolic enzymes has been observed following long-term electrical stimulation; a slow-to-fast fiber transformation is achieved with phasic high-frequency stimulation, and a fast-to-slow transition is achieved through chronic low-frequency stimulation. Chronic low-frequency stimulation decreases the surface area of the neuromuscular junctions and the postsynaptic folds. Many studies have documented an increase in type I slow-twitch fibers with endurance training, and the opposite fiber type transformation (an increase in type IIa fibers) has been shown following high-intensity, short-duration sprint training.
muscle gene expression (6). The regulation of fiber type-specific gene expression likely occurs through both the pattern of electrical activity (and the resultant calcium release) and the trophic factors released at nerve termini. For example, it has been shown that reinnervation of muscles by motor neurons with a different firing pattern results in muscle fiber transformation. Similarly, remodeling of muscle fiber type distribution, myofi-brillar proteins, mitochondria number, and metabolic enzymes has been observed following long-term electrical stimulation; a slow-to-fast fiber transformation is achieved with phasic high-frequency stimulation, and a fast-to-slow transition is achieved through chronic low-frequency stimulation. Chronic low-frequency stimulation decreases the surface area of the neuromuscular junctions and the postsynaptic folds. Many studies have documented an increase in type I slow-twitch fibers with endurance training, and the opposite fiber type transformation (an increase in type IIa fibers) has been shown following high-intensity, short-duration sprint training.
Calcineurin is a calcium/calmodulin-dependent phosphatase and is activated by sustained calcium elevation. Calcineurin is known to dephosphorylate the transcription factor nuclear activated T cells (NFAT), which enables NFAT to translocate into the nucleus and activate gene transcription in T cells. It has been proposed that calcineurin could regulate muscle-specific gene expression by transducing the different calcium signals transmitted through either tonic neural activity or intermittent bursts of neural activity. Calcium concentrations are relatively high in slow muscles and low in fast muscles. Activated calcineurin can upregulate slow fiber-specific gene promoters, and inhibiting calcineurin results in slow-to-fast fiber transformation (7). Further, the transcriptional activation of slow-fiber genes is mediated by the NFAT and MEF2 transcription factors.
CHANGES IN MUSCLE MORPHOLOGY AND FUNCTION IN DIABETES
In addition to the well-established metabolic changes in skeletal muscle associated with the progression of diabetes (see below and other chapters), there can be deleterious changes in muscle morphology associated with the advancement of this disease. It is often difficult to determine the evolution of such morphologic change because these types of alterations may precipitate from other end-organ complications, decreased activity, and/or increasing age. Some changes are observed only with long-term, uncontrolled type 1 diabetes.
A rare but severe complication of poorly controlled, long-duration type 1 diabetes is acute-onset diabetic muscle infarction (DMI). DMI is characterized by edema, tenderness, and muscle weakness and commonly presents in the vastus lateralis, thigh adductors, biceps femoris, and infrequently the triceps surae. Histologic assessments demonstrate edema; large areas of necrosis, fibrosis, regenerating fibers, and lymphocyte infiltration; and vascular thickening and thrombosis (8,9).
Characterizing the etiology and pathophysiology of type 1 diabetes through patient examination is limiting, as the physiology, genetics, and environment cannot be controlled. Several animal models of type 1 diabetes have been used to enable more comprehensive characterization and therapeutic and preventive strategies. One of the most commonly utilized models is administration of low-dose streptozotocin, which is toxic to β-cells, with diabetes ensuing after a few days (10). Animal models of diabetes have been used to study the time course of morphologic, neurologic, and vascular changes in skeletal muscle with diabetes. Diabetes progression can be associated with atrophy of skeletal muscle fibers, muscle weakness, diminished nerve activity, and decreased skeletal muscle blood flow (11,12,13). Diabetic neuropathies affect both sensory and motor nerves, as well as the autonomic nervous system. The physiologic derangements leading to neuropathies are not well defined, but it is hypothesized that hypoxia (precipitating from diabetic vasculitis) and hyperglycemia are primarily involved, as normalizing blood glucose is the most effective therapy (10). Hypoxia results in part from decreased capillary bed density and luminal diameter, resulting in diminished blood flow and oxygen delivery to the skeletal muscle (11). Alterations in the neuromuscular junction, muscle architecture, excitation-contraction coupling, and contractile properties have also been observed in streptozotocin-induced models of diabetes (12,14,15). A decrease in the number of synaptic vesicles and lower resting and end-plate potentials have been observed following induction of diabetes in mice. Further, streptozotocin-induced diabetes causes ultrastructural changes in the nerves and muscle fibers, including disrupted neurofilament and microfilament architecture and swollen and disrupted orga-nelles (15). Similarly, a decrease in twitch tension and calcium mobilization in the sarcoplasmic reticulum has been observed in streptozotocin-treated mice (12,14). Together, these alterations may significantly decrease skeletal muscle contractile function.
SKELETAL MUSCLE METABOLISM
The metabolic rate in skeletal muscle is finely regulated by the acute energy requirements of the tissue and by hormonal mechanisms. In the resting condition, the major source of fuel for skeletal muscle is circulating nonesterified free fatty acids, providing approximately 85% to 90% of the required fuel (16). In the postprandial condition, when insulin is released from the pancreas, glucose uptake into muscle increases and the free glucose is rapidly phosphorylated by hexokinase to glucose-6-phosphate. If the muscle is not actively contracting, the majority of glucose-6-phosphate is shunted toward nonoxidative glucose disposal, resulting in increased muscle glycogen. The presence of high levels of glycogen in skeletal muscle is a unique feature of the sarcoplasm compared with the cytosol of other cell types.
Skeletal muscle is also unique among tissues because of its need to respond rapidly to large changes in cellular metabolism. There is only enough ATP stored within muscle fibers to sustain contractile activity for less than 1 or 2 seconds. The first source of energy used to restore ATP is phosphocreatine, which is stored within the muscle at levels that are approximately five times higher than those of ATP. When the chemical bond between creatine and phosphate is broken, the phosphate ion is transferred to ADP to form ATP by a reversible reaction catalyzed by creatine kinase. However, phosphocreatine as an energy source for ATP regeneration is also limited, capable of supporting only a few seconds of maximal muscle contractions. Energy is then provided for the resynthesis of ATP both by the anaerobic breakdown of glucose and glycogen to pyruvate and lactate and by the aerobic oxidation of carbohydrates, lipids, and proteins. The details of these metabolic pathways, along with a thorough discussion of their interactions, are described in other chapters in this text (Chapters 8,16,17, and 24).
Fatty Acid Metabolism
Of the various lipids in the body, fatty acids are the most important metabolic fuel for skeletal muscle. Sources of fatty acids can include triglycerides stored in the muscle or triglycerides stored in adipose cells within the muscle tissue (17). However, the majority of fatty acids are derived from the uptake of circulating fatty acids bound to albumin and circulating triglycerides present in the lipid core of very-low-density lipoproteins and chylomicrons. The enzyme lipoprotein lipase (LPL) is required to hydrolyze the fatty acids from circulating lipoprotein triglyceride before fatty acid transport across the vascular endothelium can occur (17). LPL activity is much higher in slow-twitch type I fibers than in more glycolytic type II fibers. Insulin downregulates LPL activity by decreasing LPL transcription and, therefore, its abundance.
The circulating fatty acids have to be transported into the mitochondria for oxidation, passing through the vascular endothelium, the interstitial space, the sarcolemma, and finally the outer and inner mitochondrial membranes. The transport of fatty acids across the vascular endothelium has not been clearly elucidated, but it is accepted that the fatty acids diffuse through the endothelium following release from albumin and are likely to rebind to interstitial albumin and to be brought to the sarcolemmal membrane. Transport across the sarcolemmal membrane is mediated by a transmembrane fatty acid transporter (FAT) found in a variety of tissues, including fat and muscle (17), although the mechanism and kinetics of this transporter are not fully understood. Once inside the sarcoplasm, fatty acids bind to fatty acid binding protein (FABP) and diffuse across the sarcoplasmic space to the mitochondrial membrane. The fate of fatty acids within skeletal muscle is either storage, in the form of triglyceride, or oxidation. After esterification to coenzyme A (CoA) by fatty acyl CoA synthetase, activated fatty acids can be transported into the mitochondria by carnitine to be oxidized (17). Carnitine acyl transferase I in the outer mitochondrial membrane converts fatty acyl CoA to acyl carnitine, which crosses the inner membrane via the carnitine-acyl carnitine translocase, in exchange for free carnitine. In the inner mitochondrial membrane, carnitine acyl transferase II catalyzes the exchange of the carnitine in the acyl carnitine for CoA, yielding intramitochondrial fatty acyl CoA, to be oxidized through the Krebs cycle.
Obesity and type 2 diabetes are associated with increased circulating levels of fatty acids and triglycerides and decreased muscle oxidation of fatty acids, leading to the accumulation of triglycerides in muscle (18). Lipid accumulation in muscle and liver is known to impair insulin signaling and metabolic enzyme activity and is strongly linked to the development of skeletal muscle insulin resistance and the multitude of diabetic metabolic complications (19). Indeed, it has been shown that interventions such as weight loss induced by dietary restriction and physical activity or treatment with peroxisome proliferator-activator receptor-γ agonists, the thiazolidinediones, can decrease muscle triglyceride content and improve insulin sensitivity (18,19). The precise mechanisms for the decrease in oxidation of fatty acids in skeletal muscle and the increase in lipid esterification in diabetes and obese states are unclear. However, proportional decreases in carnitine palmityl transferase 1 activity, the long-chain fatty acyl CoA mitochondrial translocase, and oxidative enzymes are consistent with a reduction in mitochondrial content or function or both (20).
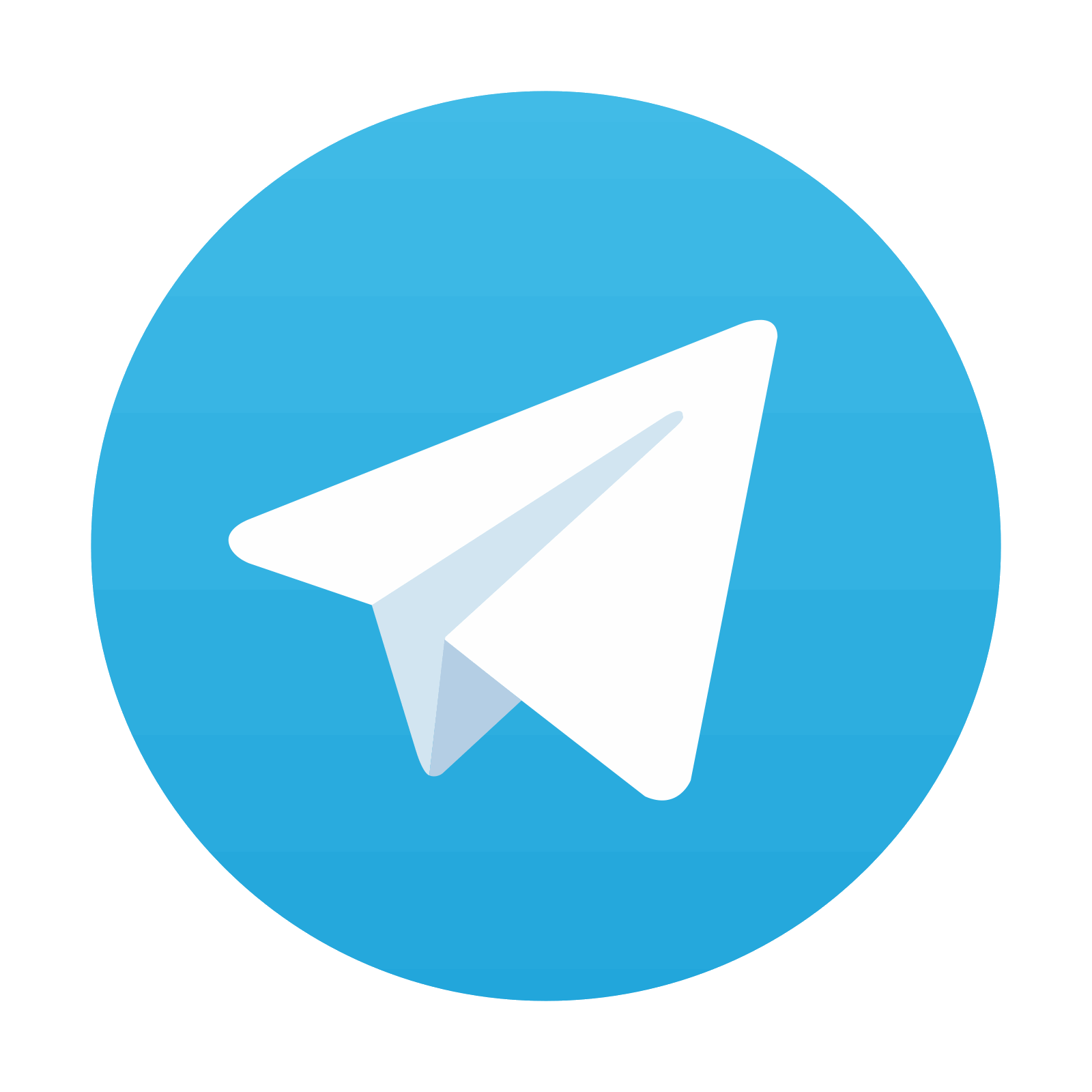
Stay updated, free articles. Join our Telegram channel
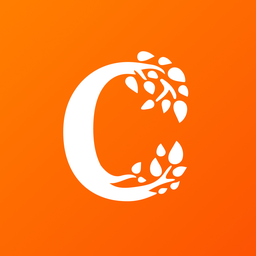
Full access? Get Clinical Tree
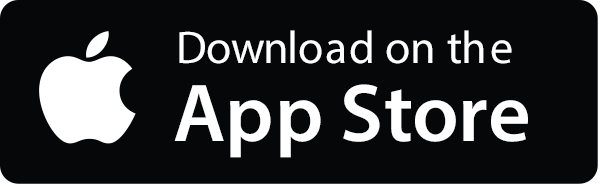
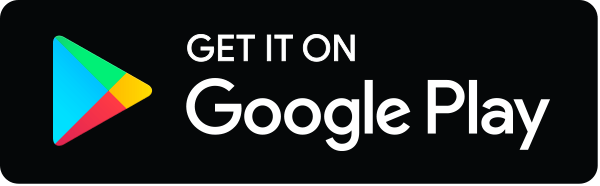