2University of Edinburgh, UK
- The response to stress involves a cascade of neuroendocrine and proinflammatory events, which interact and influence each other.
- Together, these responses can have profound catabolic effects on body metabolism, especially following major surgery or trauma.
- A series of measures needs to be taken in the surgical/traumatised patient in order to ensure an adequate provision of and tolerance for nutritional support.
- In critical illness, blood glucose levels should be controlled (if necessary with insulin) to avoid hyperglycaemia.
- No single action will be sufficient to counteract the stress of injury, but a multimodal enhanced-recovery programme should be undertaken in order to turn the patient from catabolism towards an anabolic phase and hence recovery.
20.1 Introduction
Surgery and trauma constitute a major part of modern medicine. In the Western world, every year about 5% of the population has an operation, and some 40% of patients in hospitals undergo an operation as part of their treatment. Accidental major trauma is substantially less frequent, but these patients often require intensive care for prolonged periods of time.
While elective surgery represents an elective type of treatment in which deliberate actions are undertaken to remove or reconstruct organs, and hence can be well planned, trauma represents quite a different situation. Trauma involves a variety of different injuries and affects different parts of the body in different combinations: uncontrolled injuries have occurred, the patient is in a variable state of resuscitation, and the trauma team has to adapt to the situation as best possible. The objective of treatment is to preserve as many organs and bodily functions as possible with a minimum of further trauma.
Although the injuries can be very different, the response to injury is quite similar in surgery and in accidental trauma. The difference between the two lies in the ability to prepare the patient for the injury and to control homeostasis and the stress response in the elective surgical patient, which is not possible in the traumatised patient. The fact that elective surgery care programmes can be proactive, and the surgery performed under well-controlled and planned perioperative conditions, opens the possibility of enhancing recovery after elective surgery. The situation is different in trauma, where many of the proactive initiatives cannot be undertaken, for obvious reasons. Nevertheless, the global strategy for metabolic control and nutritional support is similar in both situations.
20.2 The stress response to trauma and its effects on metabolism
It is now about 70 years since Sir David Cuthbertson introduced the terms ‘ebb’ and ‘flow’ to describe the metabolic response to long-bone fracture (Figure 20.1). His was the first attempt to introduce some chronological order into the response to trauma and to include ‘shock’ as an integral part of the response, rather than as a complication. It is important to recognise that the main features of the metabolic response are initiated at the time of the injury and that this is probably the time at which modulation will be most effective. The mediators of such change include the classical neuroendocrine hormones, along with proinflammatory mediators.
The complexity of the interaction between the different components involved in traumatically induced stress is illustrated in Figure 20.2. The injury caused by the operation initiates an inflammatory response, causing the release of cytokines and acute-phase proteins and the activation of stress hormones. The release of these mediators causes a change of metabolism into a catabolic state. However, even in situations in which the inflammatory and endocrine responses are minimised, stress metabolism can occur. This indicates that other mechanisms are likely to be involved in causing the change in metabolism following surgery. Figure 20.2 also shows that fasting before elective surgery adds to the stress, by enhancing the endocrine stress response. In patients in whom stress-induced catabolism is pronounced or prolonged, the risk of complications increases. The development of complications will further aggravate the inflammatory and endocrine stress responses, which may become a vicious circle.
Figure 20.2 Schematic overview of the sequence of events that occurs in response to surgical trauma. NEFA, non-esterified fatty acids; RQ, respiratory quotient.
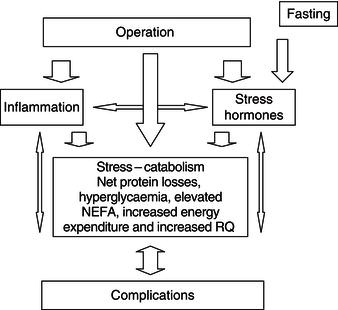
It is perhaps no longer useful to think of the ‘ebb’ phase as a period of depressed metabolism, but rather as the early stage after trauma during which tissue energy production is not limited by oxygen delivery. It is a neuroendocrine response and depends on the magnitude of blood loss and somatic afferent nervous stimuli arising from damaged tissues. These stimuli inhibit homeostatic reflexes subserving both the thermoregulatory and cardiovascular systems. After very serious injuries with failure of oxygen delivery, the ‘ebb’ phase of altered control mechanisms may be short-lived, as the patient enters into the potentially irreversible spiral of cell and tissue death known as ‘necrobiosis’. The ‘flow’ phase is characterised by an increase in body temperature, metabolic rate, and urinary nitrogen excretion, associated with net protein breakdown, a reduction in muscle mass, and disturbances in energy substrate disposal.
Hypermetabolism
While it has been accepted since Cuthbertson’s pioneering studies that hypermetabolism occurs in the ‘flow’ phase and that the increase is directly related to the severity of injury, there has been a considerable change in our understanding of the magnitude of that increase. In the early 1970s it was believed that the untreated, critically ill, septic, or trauma patient was expending in the region of 5000 kcal/day, creating major problems for those trying to introduce nutrition to the care of such patients. The introduction of clinically useful indirect calorimeters demonstrated that measured energy expenditures were most commonly less than half such predicted values. There was an understandable reluctance to accept these measured values until it was appreciated that a number of the features of critical illness and its treatment would reduce metabolic rate and therefore counterbalance those factors that tended to increase energy expenditure. Severely injured patients or those having undergone major surgery demanding intensive care will be resting in bed and hence the increase in basal metabolic rate (BMR) will be compensated for by the reduction in physical activity. Such patients may often be in bed for long periods, paralysed, ventilated, nursed in a warm environment, and receiving inadequate feeding, and will lose active muscle mass. It is, however, important to ensure that the metabolic rate is not limited by an inadequate supply of oxygen. Thus the majority of trauma patients have energy expenditures only 15–25% above their predicted healthy resting values. The exceptions may be those with excessive burns, although the introduction of early wound excision and grafting in place of treatment with exposure and delay of grafting has reduced the problem of massively elevated energy demands.
There are several factors leading to hypermetabolism and, therefore, an increase in energy expenditure in critical illness. First, there is an upward resetting of thermoregulation, which mediates an increase in energy production through enhanced activity of the sympathetic nervous system. A positive relationship has been demonstrated between urinary catecholamine excretion and metabolic rate in burn injury. Although plasma catecholamine concentrations are increased during the ‘flow’-phase response to burns and severe head injury, they are apparently normal after severe musculoskeletal injuries and sepsis. However, such plasma levels may provide only limited information about the state of sympathetic activation following trauma or sepsis as catecholamine turnover is known to double in sepsis.
An increase in sympathetic activity may stimulate metabolic rate by enhancing substrate cycling between non-esterified fatty acids and triacylglycerol, glucose, and glycolytic products. Similarly, protein–amino acid recycling may equally contribute to the increased BMR. The wound, be it an abscess, burn, or fracture, acts as an extra organ and also contributes to this glucose cycling. Lactate produced by anaerobic glycolysis in the wound is transported to the liver, where it is converted to glucose in the Cori cycle, an energy-consuming process. The wound will also contribute to hypermetabolism in a number of other ways. For example, wounds have a hyperaemic circulation, which is not under neural control, and may require an increase in cardiac output and energy expenditure. Activated inflammatory cells in the wound (and elsewhere) have a high oxygen consumption and release a number of cytokines (e.g. interleukin 1 beta (IL-1β) and tumour necrosis factor alpha (TNFα), which have been implicated in the centrally mediated upward resetting of metabolic activity.
It was believed that the increase in protein turnover associated with the ‘flow’ phase contributed at least 20% of the increase in the metabolic rate, but this is now believed to be an overestimate, except perhaps in children. Nevertheless, intravenous feeding with amino acids elevates body temperature and can be used as a way of maintaining body temperature during major surgery. There may also be an iatrogenic component to hypermetabolism, especially for those being fed parenterally.
The administration of calories (especially carbohydrates) has been shown to increase sympathetic activity and metabolic rate. This response may be enhanced in sepsis (although this has not been a universal finding). In some patients, glucose-based total parenteral nutrition (TPN) has been associated with an increase in respiratory minute ventilation greater than that expected from the increased metabolic load. This respiratory distress can be ameliorated by replacing some of the glucose with fat emulsion, thereby suggesting that increased CO2 production (most pronounced when the respiratory quotient (RQ) rises above 1.0) may be an important factor. Amino acid solutions can also increase ventilatory ‘drive’. This may be due to their low pH, but can also be secondary to a decrease in the serotoninergic suppression of ventilatory drive following inhibition of tryptophan uptake into the brain by increased levels of branched-chain amino acids in the plasma.
Thus the ‘flow’ phase of the response to trauma/sepsis is characterised by a moderate degree of hypermetabolism. A number of factors mediate the increase, but the most important seems to be a complex interaction between the wound and the central control of metabolic rate, in which the cytokines may have a pivotal role. The proinflammatory response, in combination with counter-regulatory hormones, may also mediate the changes in peripheral metabolic control, including insulin resistance.
Protein metabolism
Cuthbertson noted an increase in urinary nitrogen excretion after long-bone fracture, which he attributed to increased protein breakdown. A recent study showed that following either severe trauma or sepsis, patients admitted to intensive care sustained, on average, a loss of 17% of body water, 16% of total body protein, and 19% of total body potassium over 21 days. These losses were greater than can be accounted for by bed rest alone and occurred even when the patient was in a positive energy balance. The major site of protein loss was skeletal muscle but nitrogen loss also occurred in respiratory muscles and in the gut, although cardiac muscle appeared to be mostly spared.
Muscle wasting represents an imbalance between protein synthesis and degradation. After minor/moderate surgery there is a decrease in synthesis, mirroring the response to short-term starvation. With a more severe insult, protein synthesis in different tissues can be increased (for example, acute-phase protein synthesis in the liver) or decreased (muscle protein synthesis), while protein degradation in skeletal muscle is increased. Inflammatory conditions are associated with an increase in the hepatic synthesis and plasma concentration of a number of so-called positive acute-phase proteins. The positive acute-phase proteins include fibrinogen, C-reactive protein, alpha-1-acid glycoprotein, haptoglobulin, alpha-1-antitrypsin, alpha-2-macroglobulin, and ceruloplasmin. In the human hepatocyte, the main proinflammatory cytokine regulating this response is interleukin-6 (IL-6). On the other hand, the plasma concentration of a number of liver export proteins (e.g. albumin) falls acutely (negative acute-phase reactants) after injury.
Rather than reflecting a reduced synthesis rate, the fall in the plasma concentration of negative acute-phase proteins is thought to be due mainly to increased transcapillary escape, secondary to an increase in microvascular permeability. Thus, although the plasma concentration of the negative acute-phase reactants falls, the net metabolic demands on the liver increase due to continued synthesis of negative reactants, combined with increased synthesis of positive reactants.
The increased protein degradation that occurs in skeletal muscle following injury may be due to increased activity of three different systems: the lysosomal system, the Ca2+-dependent calpain pathway, and the ubiquitin–proteasome pathway. Recent research has suggested that the ubiquitin–proteasome pathway is the major pathway but that it works in concert with the calpain system. The metabolic significance of increased activity of the ubiquitin–proteasome pathway comes from the fact that the process whereby proteins are tagged with ubiquitin for degradation in the proteasome requires adenosine triphosphate (ATP) and therefore, once activated, this pathway not only contributes to the negative nitrogen balance but also the negative energy balance of the patient.
Insulin resistance
Insulin is the best-known anabolic hormone. It is normally released promptly in response to feeding and ensures the storage of nutrients. Although insulin levels peak within less than an hour after feeding, the effects on metabolism remain for another few hours. Insulin promotes oxidation and storage of carbohydrates and fat, and it has a protein-sparing effect.
Insulin resistance is commonly associated with diabetes mellitus. However, in many situations of stress, such as accidental trauma, sepsis, burns, and elective surgery, a state of transient insulin resistance develops in otherwise healthy individuals. From a theoretical point of view, many of the metabolic changes found following trauma can be explained by a reduction in the effectiveness of insulin in exerting its normal actions. In most clinical situations of stress, the resistance to insulin can be overcome by insulin treatment. This was shown to be of importance in postoperative patients in need of ventilatory support in the intensive-care unit (ICU). When glucose levels were maintained at normal levels, morbidity was markedly reduced and mortality almost halved. Interestingly, the effects of insulin were a reduction in a series of complications usually associated with chronic diabetes, such as sepsis and infections, renal function, and polyneuropathy. It is well known that these complications occur in the ICU, and they are clearly associated with poor metabolic control. While the first studies using intensive insulin treatment had very good results when aiming at normoglycaemia, large-scale follow-up studies in general ICU patients and in postoperative patients revealed risks of adverse outcomes due to hypoglycaemia. Therefore, the target glucose level for this treatment in the ICU has been modified from strict normoglycaemia (4.5–6.0 mmol/l) to a moderate hyperglycaemia of 7–8 mmol/l in order to avoid the risks associated with hypoglycaemia.
Insulin resistance in elective surgery has also been studied in detail. The degree of insulin resistance that develops is related to the magnitude of the operation. Following routine upper-abdominal surgery, insulin resistance prevails for about 2–3 weeks. Surgical technique is also a factor affecting the magnitude of insulin resistance, in that laparoscopic techniques result in less pronounced insulin resistance than open surgery. Many of the changes in glucose metabolism following surgery are similar to those found in type 2 diabetic subjects (Table 20.1
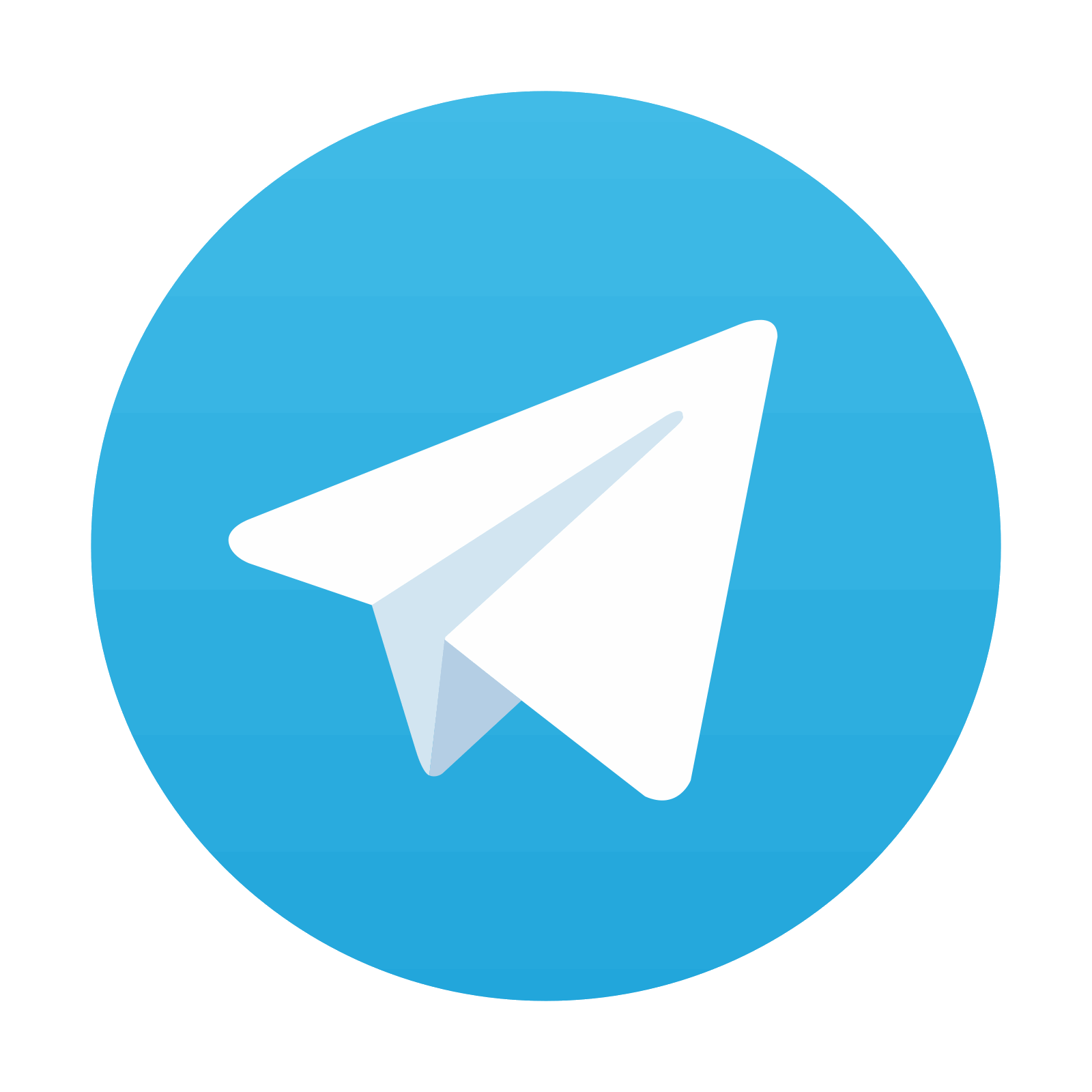
Stay updated, free articles. Join our Telegram channel
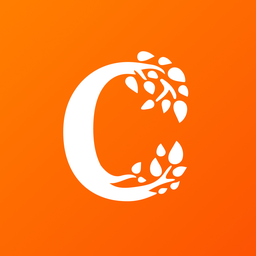
Full access? Get Clinical Tree
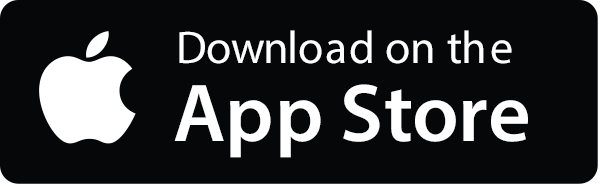
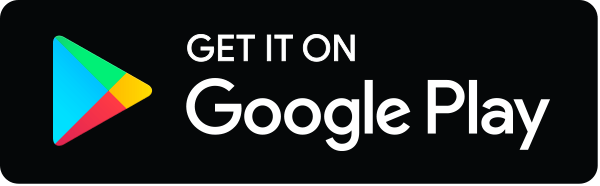