Modeling therapy of late or early-stage metastatic disease in mice
Robert S. Kerbel, PhD Marta Paez-Ribes, PhD
Shan Man, BSc
Ping Xu, PhD
Eric Guerin, MD
William Cruz-Munoz, PhD
John M. L. Ebos, PhD
Overview
An ongoing problem in oncology drug development is the frequent failure of preclinical therapy models involving treatment of tumor-bearing mice, which show positive results, to predict similar success in patients enrolled in clinical trials. There are numerous possible reasons for causing such high rates of false positives. One is the failure to reproduce the clinical circumstances of treating systemic metastatic disease, whether microscopic or macroscopic in nature—but especially the latter. Thus, it is still common practice to treat mice with established primary tumors, whether they are transplanted or spontaneous, of mouse or human origin, or derived from cell lines or tumor tissue grafts—including human patient-derived xenografts (PDXs). In this chapter, we summarize recent progress in developing mouse models of spontaneous metastases, especially of late-stage disease, after surgical resection of primary tumors, including the use of human tumor cell lines, PDXs, and genetically engineered mouse models (GEMMs). Some limited therapy results using such models, and how they retrospectively or prospectively correlated with relevant phase III clinical trial outcomes, are discussed. A limited database indicates the possible benefits of using such models for predictive investigational therapeutic studies relevant to the treatment of patients with metastatic disease. Some limitations of such models are also discussed.
Introduction
The development of new cancer drugs is a painstakingly complex, laborious, and expensive undertaking, associated with an ultimate failure rate much higher than any other therapeutic area; indeed, it has been reported that greater than 60% of all phase III trials in oncology fail to meet their primary endpoint and are thus considered failures.1 Such long-term and hugely expensive trials almost invariably follow what were highly encouraging preclinical results—usually undertaken in mice—as well as subsequent small phase I and II clinical trials that also suggested encouraging results, thus bolstering the decision to proceed with further development at the pivotal double-blind placebo-controlled randomized phase III clinical trial level. The subsequent high failure rate at the phase III trial level has therefore resulted in a number of critical questions, and among them include the following: (1) what are the reasons for the tendency of preclinical models involving therapy of tumors in mice to overpredict encouraging outcomes, and how might such models be improved for predicting future clinical trial outcomes? (2) what are the reasons for the failure to reproduce positive results detected in phase II clinical trials in subsequent phase III trials—and how might the design of such smaller phase II trials be improved to better predict what will happen at the phase III level?
This chapter will focus with the first question beginning with a discussion of the numerous possible factors that lead to highly exaggerated “false positives” of therapeutic outcomes obtained in mouse therapy studies, followed by an analysis of some strategies designed to overcome current mouse tumor therapy model limitations. Special emphasis will be placed on one particular approach to improve clinical relevance of mouse tumor models, namely, recapitulating clinical treatment of systemic metastatic disease, early (microscopic) or (overt) late stage, especially the latter.
Some factors that reduce the clinical relevance and hence translatability of most mouse tumor therapy models
Many reviews or commentaries have been published on the limitations of preclinical mouse tumor therapy models for assessing cancer drug activity, at least with respect to clinical relevance and predictive potential.2–4 Some factors are well known, but others less so, and hence less appreciated. Among them include the following: (1) it is virtually unknown for therapy studies in mice to be initiated in older animals that would be equivalent to the age of the majority of cancer patients, that is, middle age or elderly.5 Instead, it is typical for therapy to be initiated in mice (at least when using transplanted tumor models) at 6–10 weeks of age. As such, this would be the roughly equivalent of undertaking a pediatric oncology clinical trial. However, it is well known that the impact of cancer drugs in children can be vastly different when compared to adults because of critical parameters that impact cancer drug activity such as drug metabolism and pharmacokinetics; (2) in contrast to a typical clinical trial where every patient enrolled is genetically distinct, mouse studies using inbred mouse strains obviously involve a high degree of homogeneity with respect to the population under study. This essentially eliminates or severely reduces the impact in humans of pharmacogenomic heterogeneity on such vital parameters as drug half life and metabolism, which can strongly impact therapeutic outcomes6; (3) either the optimal, or maximum tolerated doses (MTDs) of drugs can be very different between mice and humans; for example, the MTD of many different chemotherapy drugs may be much higher in a mouse compared to humans.6 This can cause potent tumor response artifacts, especially when using human tumor xenografts for the therapeutic studies; (4) many commonly used transplanted mouse tumor models are highly immunogenic and as a result, this can facilitate the immune system (provided an immunocompetent mouse is used as a host for the therapeutic investigations) to have an influence on therapeutic outcome—but in a way that could distort or exaggerate the degree of efficacy obtained7; (5) the endpoints used in preclinical studies are often clinically irrelevant or inappropriate.8 For example, a common clinical endpoint, such as progression free survival (PFS) based on imaging, is rarely used to assess efficacy in mice and yet PFS is increasingly used as a primary or secondary endpoint in most phase III clinical trials. Instead, it is typical, especially when using tumor transplants, to simply detect an effect on tumor growth per se, usually involving established primary tumors. A growth delay is often noted in such studies in the absence of any overt tumor regression. From a clinical perspective, growth delays would be viewed as “treatment failure”/progressive disease, and yet such results are often viewed as encouraging by cancer researchers because there is a nontreated control group allowing the benefit between the treated and untreated groups to be observed; (6) as any quick glance through current cancer research journals will indicate, the main way cancer drugs are tested in mice remains very much the same as what was half a century or more ago: tumor cells are transplanted into mice, often subcutaneously, to establish a “primary” ectopic tumor, which is then “staged” to a certain size, and treatment of the tumor-bearing mice subsequently initiated. These transplanted tumors can be of either mouse or human origin. In both cases, especially when using mouse tumors, doubling times can be extremely short owing to a very high tumor cell proliferative rate. This can render the tumor hypersensitive to various cancer drugs, especially cytotoxic chemotherapeutic agents that preferentially target cells in cycle replicating DNA or activating intracellular machinery such as microtubules or various enzymes involved in DNA replication. Moreover, tumor cells in cycle might also have an impact on their sensitivity to other types of drug such as signal transduction inhibitors. However, such high cell proliferative rates are not typical of most spontaneous human cancers; (7) the clinical circumstance of treating advanced systemic disease in multiple organ sites such as the lungs, liver, brain, and bones is rarely undertaken in preclinical mouse model studies.4 Compared to therapy of primary tumors in mice—be they transplanted or spontaneously arising—treating mice with overt metastatic disease is more cumbersome, expensive, and time consuming. However, it is well known that late-stage systemic disease in humans in common solid malignancies is difficult to successfully treat—indeed, it is essentially incurable and associated with very modest gains in PFS or overall survival in “positive” phase III clinical trials. Such gains may be statistically significant and associated with a respectable hazard ratio—but the clinical meaningfulness of such results and their associated cost effectiveness are often marginal.9
What follows is a discussion of some of the current attempts to model therapy of metastatic disease in mice; with the emphasis on advanced-stage disease, and also some comment is provided on early-stage (micrometastatic) disease, utilizing transplanted tumors established from either human tumor cell lines or patient-derived xenografts (PDXs) as well as spontaneously arising tumors in genetically engineered mouse models (GEMMs) of cancer.
On the development of postsurgical human tumor xenograft models of advanced visceral metastatic disease using established cell lines
Beginning about a decade ago, attempts were made to develop models of advanced “spontaneous” metastatic disease from resected established primary tumors, virtually the first of their kind.10 The basic methodology employed involved a variation of the pioneering studies of Fidler11 who first reported the derivation of variant sublines from the B16 mouse melanoma capable of more aggressive metastatic spread, especially to the lungs, after serial selection in vivo involving successive intravenous tumor cell inoculations and recovery of resultant “artificial” or “experimental” lung metastases. In these studies, 10 successive intravenous-mediated cell selections were undertaken, thus generating successive sublines of increasing metastatic properties after intravenous inoculation, for example, the B16F1 and B16F10 sublines.11 In our case, we made an attempt to select similar variants capable of spontaneous metastatic spread from an established, but resected orthotopic primary tumor10 so that the metastatic cells would need to accomplish all of the early and late steps necessary for establishment of a distant metastasis. To achieve this, an established human breast cancer cell line, for example, the “triple-negative” MBA-MD-231 cell line, was implanted into the mammary fat pads of female immune deficient (SCID) mice, a procedure known to promote the rate and extent of distant metastatic spread to sites such as the lungs, compared to ectopic injection of cells into the subcutaneous space.12, 13 Once the primary tumors attained a predesignated volume/size, for example, 500 mm3, resection was undertaken. This was considered necessary to allow the mice to survive for a longer period of time, thus increasing the probability that tumor cells seeded from the primary tumor would have sufficient time to establish macroscopic metastases in distant organs, for example, the lungs. Such metastases could be detected by gross inspection beginning after a period of about 4 months, or longer, post surgical resection of the primary tumors.10 Several such lung metastases were harvested and a cell line established from the pooled population of metastases. These cells were then reinjected once more into the mammary fat pads and the subsequent primary tumors resected approximately 4 weeks later. This resulted in an accelerated rate and degree of pulmonary metastases (and sometimes, variably, extrapulmonary metastases). An individual lung metastasis was harvested and a subline established from it, for example, a more aggressive metastatic variant called LM2.4.10 It would then be used for experimental therapeutic studies by once again injecting the cells orthotopically, resecting the resultant primary tumors, allowing the mice to develop overt metastatic disease, which now occurred within about 1 month—mostly confined to the lungs but sometimes involving other sites such as the lymph nodes or liver.10 It would be at such a late stage of tumor progression that an experimental therapy would be initiated, with survival used as a primary endpoint for assessing therapeutic efficacy.10 In addition, such variant pro-metastatic sublines can be tagged with markers to monitor disease burden and response to therapy over time. Such markers include luciferase that permits whole body optical/bioilluminscent imaging14 as well as undertaking transduction of the gene for the β subunit of human choriogonadotropic protein (β-hCG), levels of which can be detected in urine as a molecular surrogate of tumor burden.15 These same procedures have been successfully employed to develop models of advanced metastatic disease not only for human breast cancer but also for human malignant melanoma,16 renal cell carcinoma,17 and colorectal carcinoma.18 In addition, models of advanced intraperitoneal metastatic ovarian cancer had been developed, which did not involve prior serial selection in vivo19 as well as locally advanced orthotopic hepatocellular carcinoma.20
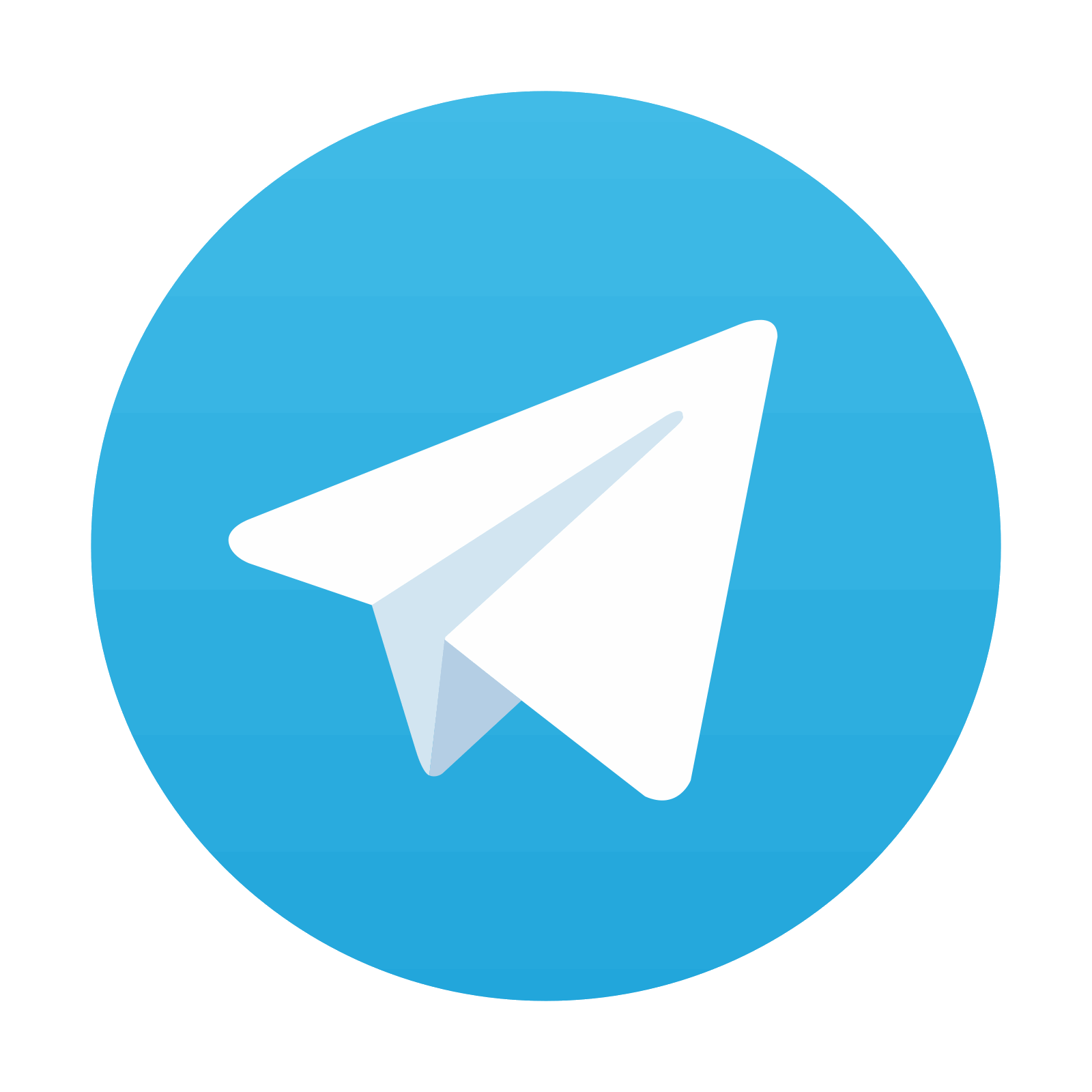
Stay updated, free articles. Join our Telegram channel
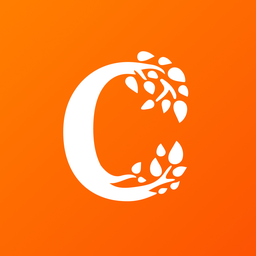
Full access? Get Clinical Tree
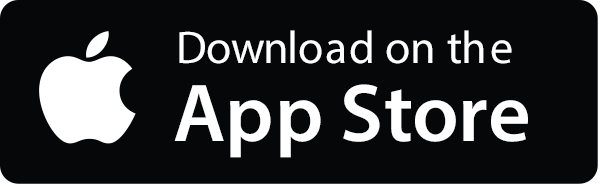
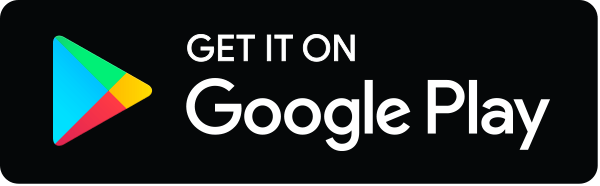