Mechanisms of Diabetic Microvascular Complications
Christian Rask-Madsen
Zhiheng He
George L. King
Patients receiving modern care for diabetes have a dramatically lower risk for developing microvascular complications than they did a few decades ago. In particular, the risk for developing renal disease necessitating dialysis has decreased severalfold. However, diabetes is still the most common cause of blindness and end-stage renal disease and a major reason for peripheral neuropathy. Therefore, there is compelling reason to increase our knowledge about the cellular and molecular mechanisms of these complications so that rational strategies for prevention and treatment can be designed.
Microvascular complications in diabetes have traditionally included retinopathy, nephropathy, and neuropathy. In each of these complications, pathologic changes and cellular dysfunction are seen in nonvascular tissues at early stages and cannot be explained by circulatory changes alone. Thus, in retinopathy and neuropathy, dysfunction of neurons develops in parallel with microvascular pathology. Therefore, these complications cannot be explained entirely as being secondary to microvascular pathology. Nevertheless, this chapter will focus on vascular mechanisms for the development of these complications.
Cardiovascular disease is the major reason for morbidity and mortality among patients with diabetes. Many aspects of heart disease in patients with diabetes are secondary to atherosclerosis, i.e., macrovascular disease. But the mechanisms of some of the myocardial pathologies in diabetes have obvious similarities to mechanisms of complications in other organs, e.g, in the kidney. Therefore, in this chapter we have chosen to describe changes in regulation of vascularization and extracellular matrix in the heart.
Many of the disease mechanisms described below have been demonstrated in several tissues and cell types in diabetes; e.g., oxidative stress, glycation, and activation of protein kinase C (PKC) have been described in all tissues affected by microvascular complications. Therefore, the later sections of this chapter discuss such mechanisms without systematically referring to where they operate. However, one of the biggest challenges in studying these mechanisms is to understand why certain tissues or cell types are preferentially affected by diabetes. For example, even though oxidative stress or activation of PKC occurs in both capillary endothelial cells and pericytes in the retina, there is a pronounced loss of pericytes rather than endothelial cells. Treatment with new drugs, especially those administered systemically, will also have to consider such tissue differences. For example, inhibitors of vascular endothelial growth factor (VEGF) have been proposed as therapy for proliferative retinopathy, but even though increased retinal expression of VEGF may be partly responsible for this complication, decreased expression of VEGF in the heart may be responsible for decreased vascularization of the ischemic myocardium in diabetes (1). Likewise, speculations that inhibition of renal expression of transforming growth factor-β (TGF-β) may prevent renal disease (2,3) should consider the possible importance of TGF-β for the development of collateral vessels to ischemic tissue (4).
The studies discussed below have used many methodologies. When interpreting results from studies in animals or in vitro, one should keep certain issues in mind. Animal models of nondiabetic insulin resistance, type 1 diabetes, and type 2 diabetes exist. They may be different from human diabetes for a variety of reasons: species differences; the introduction of hyperglycemia by a diet that is not typical for either the animal or humans (i.e., rich in galactose); induction of diabetes with a toxin given systemically (i.e., streptozotocin); the existence of a monogenic defect (mutations in the gene for leptin or the leptin receptor).
Cell-culture studies have limitations for explaining human disease. However, major advances in the understanding of diabetic complications have derived from the study of differentiated cells that preserve many of their characteristics in vitro
CHANGES IN VASCULAR MORPHOLOGY AND FUNCTION
General Vascular Changes
GROWTH AND APOPTOSIS OF VASCULAR CELLS
In proliferative retinopathy, growth of endothelial cells is evident, whereas in diabetic nephropathy or in the microvessels of the diabetic heart, loss of endothelial cells is observed. On the other hand, the contractile cells of the retinal microvasculature, the retinal capillary pericytes, are lost in diabetic retinopathy, but proliferation of vascular smooth muscle cells (VSMCs) is increased in atherosclerotic lesions.
In retinal capillaries from patients with diabetes, apoptotic pathways are preferentially activated in pericytes rather than in endothelial cells (5,6). Apoptosis in pericytes can be induced by feeding with galactose (7) and by both high glucose concentrations (6) and advanced glycation end-products (AGEs) (8).
In heart biopsy specimens from patients with diabetes, apoptosis of endothelial cells and cardiomyocytes is increased severalfold (9). In endothelial cells cultured in high glucose concentrations, the signaling pathways mediating apoptosis involve hydrogen peroxide (10), nuclear factor-κB (NF-κB) (6), and c-jun N-terminal kinase 1 (JNK1) (10).
BASEMENT MEMBRANE AND EXTRACELLULAR MATRIX
A classic morphologic finding in diabetic microangiopathy is the thickening of basement membranes (11). This phenomenon is generalized and affects both vascular and nonvascular tissues. Basement membranes maintain tissue architecture, modify cellular functions such as proliferation, and provide a filtration barrier. Basement membranes separate cells from the interstitial space or from cells of a different type. In the glomerulus of the kidney, the basement membrane is situated between endothelial cells of the capillaries and epithelial cells of the Bowman capsule; in the retina, the basement membrane separates capillary endothelial cells and pericytes. Basement-membrane proteins are synthesized by several cell types. Thus, renal mesangial matrix proteins are synthesized both by mesangial cells and by endothelial cells. The morphology of the capillary basement membrane varies in different tissues, and its thickness often correlates with the intracapillary pressure. The chemical components of the basement membrane include collagens (mainly type IV), chondroitin, heparan sulfate proteoglycans, and various glycoproteins such as laminin (12,13,14).
The capillary basement membrane in the retina thickens with age (15) but at an accelerated rate in patients with diabetes and in animal models of diabetes (16). In the retinas of diabetic rats, capillary thickening is more prominent in the inner capillary bed (nerve and ganglion layer) than in the inner and outer plexiform layers (17). In the kidney, increased extracellular matrix manifests as thickening of the glomerular basement membrane, expansion of the mesangium, and tubolointerstitial fibrosis (14). The glomerular basement membrane and the mesangium have been studied most thoroughly. In these tissue compartments, both increased synthesis of extracellular matrix proteins and decreased degradation of matrix protein have been demonstrated. Some extracellular proteins that are not a significant part of normal extracellular matrix are expressed in diabetes. However, most extracellular proteins overexpressed in diabetes are components of the normal mesangium and basement membrane. Collagen type IV and fibronectin are among the proteins most consistently demonstrated to be overexpressed in diabetes (14).
Heparan sulfate proteoglycans constitute a charge barrier to protein diffusion across the glomerular basement membrane. The majority of heparan sulfate anionic sites are in the lamina rara externa of the basement membrane (18). There is a decrease in the heparan sulfate content of the glomerular basement membrane relative to the collagen content (19). Furthermore, the number of anionic sites is decreased (18).
Expression of extracellular matrix protein is increased in diabetes. In the glomeruli, important mediators of this increased production include, among others, angiotensin II (ATII), TGF-β, and connective tissue growth factor (CTGF) (14). The roles of these mediators are discussed below. In addition, degradation of extracellular matrix protein, catalyzed by matrix metalloproteinase (MMP), is decreased. The expression of mRNA of several MMPs is reduced in patients with diabetes and in animal models of diabetes (14). Furthermore, this effect is recapitulated in cell-culture models of a diabetic milieu. For example, expression of MMP2 is decreased in mesangial cells stimulated with ATII (20). The activity of MMP is reduced by several mechanisms. MMP proenzymes are cleaved by plasmin or in the case of MMP2, by membrane-type MMP1 to yield the active form of the enzyme. The activity of both plasmin and membrane-type MMP1 is reduced in diabetes (21). Furthermore, tissue inhibitor of MMP1 is increased in diabetes (22).
ENDOTHELIAL FUNCTION
Apart from being regulated by the autonomic nervous system and circulating hormones, blood flow is controlled by substances released from the endothelium, acting in a paracrine manner. In both types of diabetes, endothelium-dependent vasodilation is decreased. Endothelial dysfunction can be seen after relatively short periods of elevated concentrations of glucose. Thus, after 6 hours of a high glucose concentration, endothelium-dependent vasorelaxation is decreased in rabbit aorta ex vivo (23) or in the forearm of healthy humans (24).
Nitric oxide (NO) is an important mediator of endothelium-dependent vasodilation, but relatively few studies have quantified how much NO contributes to blood flow during basal or stimulated conditions in tissues prone to microvascular complications. When choroidal blood flow in the cat is stimulated by facial nerve stimulation, the increase in blood flow is completely dependent on NO (25). In humans, retinal blood flow at basal conditions and stimulated by flicker is dependent in part on NO (26). Loss of NO-mediated blood flow may underlie the decrease in retinal blood flow observed in diabetes (27). Nerve blood flow in experimental diabetes is associated with a decreased contribution of NO to basal vascular tone (28).
On the other hand, vascular leakage in early streptozotocin-induced diabetes appears dependent on increased production of NO (29). This may be caused by increased expression of endothelial nitric oxide synthase (eNOS) (30,31,32) or induction of inducible NOS (iNOS) (33). Paradoxically, increased expression of eNOS or iNOS could be associated with decreased bioavailability of NO through the reaction of NO with superoxide, resulting in retinal damage through protein nitration (29,33).
Tissue-Specific Vascular Changes
VASCULAR CHANGES IN THE RETINA
Diabetic retinopathy is the leading cause of blindness in industrialized nations (34). Pathologic changes in retinal microvessel structure and function have been considered the major cause of
diabetic retinopathy (35,36). Structural changes include thickening of the capillary basement membrane, increased vessel permeability, loss of retinal pericytes, and formation of capillary microaneurysms. These structural changes are accompanied by decreased retinal blood flow, capillary occlusion, angiogenesis, hemorrhage, fibrotic tissue formation, and tractional retinal detachment. Some of these events, or all of them in combination, can ultimately result in impairment or complete loss of vision (35).
diabetic retinopathy (35,36). Structural changes include thickening of the capillary basement membrane, increased vessel permeability, loss of retinal pericytes, and formation of capillary microaneurysms. These structural changes are accompanied by decreased retinal blood flow, capillary occlusion, angiogenesis, hemorrhage, fibrotic tissue formation, and tractional retinal detachment. Some of these events, or all of them in combination, can ultimately result in impairment or complete loss of vision (35).
In physiologic states, retinal capillaries consist of contractile pericytes and endothelial cells at an approximate ratio of 1:1, which is lower than the ratio of contractile cells relative to endothelial cells in other vascular beds in the systemic circulation (37,38). This ratio drops to 1:10 in moderate-to-severe stages of nonproliferative retinopathy (39,40). Areas of pericyte loss are generally associated with microaneurysms, where endothelial cells are not supported by pericytes. It has been proposed that there are extensive interactions between pericytes and capillary endothelial cells and that the presence of pericytes is necessary for stabilization of endothelial cells and the maintenance of integrity of the vasculature (41,42,43). Loss of pericytes causes the formation of microaneurysms and acellular capillaries, further contributing to increased permeability, decreased retinal blood flow, and leukostasis (41,42,43). The role of pericytes in the integrity of retinal capillaries has been confirmed by studies of mice null for the platelet-derived growth factor-β (PDGF-β) receptor. These animals show loss of pericytes, the formation of microaneurysms, and retinal hemorrhage similar to the changes seen in diabetic retinopathy (44).
Retinal hypoxia increases the expression of a host of angiogenic factors, including, among others, VEGF and placenta growth factor (PIGF), that in turn promote neovascularization. The mechanisms for the altered expression of these growth factors and their molecular effects are discussed in later sections of this chapter.
THE RENAL VASCULATURE IN DIABETES
Acute glomerular hypertrophy occurs early in the course of diabetes (45,46), but with the progressive increase in the mesangial matrix, there is a loss in capillary surface area and thus a decrease in filtration area. Changes in the composition of the basement membrane result in altered permeability and accumulation of glomerular extracellular matrix, eventually leading to glomerular occlusion, fibrosis, and deceased filtering capacity.
The glomerular basement membrane is continuous with the tubular basement membrane via the Bowman capsule. Thickening of basement membrane and expansion of the mesangium are the dominant morphologic features in early diabetic renal disease. Thickening of the glomerular basement membrane was first described by Kimmelstiel and Wilson (47). The hemodynamic factors implicated in the pathogenesis of diabetic nephropathy include increased systemic and intraglomerular pressure. Permeability of the capillaries is altered such that the excretion of proteins with molecular masses of 44 to 150 kDa is increased. Thus, albumin excretion is increased early in diabetes and, like an elevated glomerular filtration rate, can be normalized by intensive insulin treatment (48) or islet cell transplantation (49). This initial alteration in permeability seems to be due primarily to increased filtration pressures across the glomerulus, but altered electrical-charge selectivity of the permeability barrier—changes that permit an increase in the leakage of plasma proteins—may also be involved (50).
Changes in glomerular hemodynamics are mediated in part by circulating or paracrine factors such as ATII and endothelin-1 (ET-1) (51). Similarly, several mediators lead to increased production of extracellular matrix, most notably TGF-β and CTGF. As in changes observed in diabetic retinopathy, increased vascular permeability is also an early manifestation of diabetic nephropathy, with VEGF playing an important role. Signaling pathways operating in diabetic nephropathy are discussed further in subsequent sections.
VASCULAR CHANGES IN NERVES
Diabetic neuropathy is common in diabetic patients, with a prevalence of more than 50% (52). The pathogenesis is multifactorial and considered to be both hyperglycemia-induced pathologic changes intrinsic to neurons (53) and ischemia-induced neuronal damage by decreased neurovascular blood flow (54). Because of the vascular elements, diabetic neuropathy is considered a microvascular complication. Histologically, increases in endothelial cell area and luminar narrowing of capillaries are present in the endoneurium of patients with diabetes (55). Supporting a role for decreased neural blood flow was the finding that, in rats with streptozotocin-induced diabetes, in vivo gene transfer of VEGF restored blood flow in the neurotrophic blood vessels and improved nerve function (56). A role for PKC activation in the microvascular changes in diabetic nerve was demonstrated in the same animal model, in which an inhibitor of PKCβ prevented diabetes-induced impairment of nerve blood flow (57,58).
MICROVASCULAR CHANGES IN THE HEART
Patients with diabetes have a high prevalence of chronic heart failure (59) and a high incidence of heart failure and re-infarction after acute myocardial infarction (60); these cannot be explained by more extensive coronary atherosclerosis, a higher prevalence of hypertension, or larger infarcts in this population than in people without diabetes. The causes are likely diastolic dysfunction (61) and insufficient neovascularization during myocardial ischemia (62). Myocardial disease is not a microvascular disease in the traditional sense, yet there are conspicuous similarities in the diabetic heart and traditional microvascular complications, on both pathoanatomic and molecular levels, especially with regard to the production of extracellular matrix as in the diabetic kidney.
An autopsy study showed that the capillary density in nondiabetic patients with a previous myocardial infarction was higher than in normal hearts, but capillary density in diabetic patients with a previous myocardial infarction was lower than in normal hearts (63). Similar observations have been made in animal models of diabetes (64,65), and increased capillary permeability has been reported (66). Cardiac angiogenesis and collateral formation are governed by the balanced action of a wide spectrum of pro-angiogenic and anti-angiogenic factors, including VEGF, fibro-blast growth factor (FGF), PDGF, and angiopoietins (67). The altered expression and action of these growth factors is discussed in sections below. Regardless of the individual contribution of these factors to insufficient circulation in diabetic heart disease, clinical trials aimed at treating myocardial ischemia with transfer of certain of these growth factor genes show promise, albeit they have not yet yielded definite results (67).
MECHANISMS FOR MICROVASCULAR PATHOLOGY IN DIABETES
The partial loss of insulin effects—due to dysfunction or destruction of β-cells or to peripheral insulin resistance—and the
ensuing hyperglycemia and other disturbances of metabolism have profound consequences on cellular function, extracellular matrix, organ function, and whole-body physiology. The discussion below starts with a description of systemic factors affecting the tissues prone to microvascular complications. On a cellular level, striking similarities exist between many mechanisms thought to be responsible for microvascular complications. Therefore, most of the discussion will be organized according to metabolic pathways or intracellular or extracellular signaling pathways altered by diabetes rather than being divided into sections concerned with the changes characteristic for tissues afflicted by microvascular complications.
ensuing hyperglycemia and other disturbances of metabolism have profound consequences on cellular function, extracellular matrix, organ function, and whole-body physiology. The discussion below starts with a description of systemic factors affecting the tissues prone to microvascular complications. On a cellular level, striking similarities exist between many mechanisms thought to be responsible for microvascular complications. Therefore, most of the discussion will be organized according to metabolic pathways or intracellular or extracellular signaling pathways altered by diabetes rather than being divided into sections concerned with the changes characteristic for tissues afflicted by microvascular complications.
Local Changes Caused by Systemic Factors
HYPERTENSION
Microvascular dysfunction and pathology may be initiated by hypertension. For example, the glomerular hyperfiltration observed in diabetes may be caused in part by systemic hypertension. Mechanisms have been described that link mechanical forces to vascular dysfunction, including pressure (71), stretch (72), and shear stress (73). Indirect proof that hypertension in itself may be a cause of microvascular complications is given by clinical trials of antihypertensive drugs that prevent diabetic nephropathy regardless of whether they are diuretics, calcium-channel blockers, or angiotensin-converting enzyme (ACE) inhibitors.
However, hypertension per se is not likely sufficient for the development of microvascular complications. In rats with streptozotocin-induced diabetes or in rats heterozygous for a renin transgene, endothelin receptor and angiotensin receptor 1 (AT1) antagonists both normalized hypertension and partly attenuated declining glomerular filtration rate, but only the AT1 antagonist affected renal pathology as well as expression of TGF-β and type IV collagen messenger RNA (mRNA) (74).
SYMPATHETIC NERVOUS SYSTEM
Overactivity of the sympathetic nervous system can be a participating factor in the development of hypertension in both types of diabetes (75). Furthermore, insulin is thought to have vasoconstrictor effects through activation of the sympathetic nervous system, but insulin-stimulated sympathetic activation is altered in states of insulin resistance (76). During late stages of diabetes, sympathetic denervation may alter vascular responses (76). How sympathetic overactivity, insulin resistance, and autonomic dysfunction quantitatively contribute to integrated vascular function in diabetes is unknown.
ADVANCED GLYCATION END-PRODUCTS
Modification of extracellular and intracellular proteins by sugars can result in the formation of AGEs, which is virtually irreversible (77). This reaction can take place nonenzymatically between glucose and protein through the Amadori product (1-amino-1-deoxyfructose adducts to lysine). However, much faster reactions occur between proteins and intracellularly formed dicarbonyls, including 3-deoxyglucosone, glyoxal, and methylglyoxal. These processes are accelerated by reactive oxygen species (78), perhaps by inhibition of glyceraldehyde-phosphate dehydrogenase (GAPDH), leading to an increase in the formation of triose phosphate, in turn increasing the production of methylglyoxal (79). The most prevalent AGE is carboxymethyl-lysine (80,81). Further nonenzymatic modification results in cross-linking of proteins. Because of their long turnover rate, structural extracellular proteins such as collagen are particularly susceptible to AGE modification. AGEs have been demonstrated in numerous tissues in both types of diabetes, such as the retina (82) and the glomeruli (83).
AGEs may interfere with vascular signaling by facilitating breakdown of NO (84). Furthermore, AGEs may impair the function of intracellular proteins, as in the case of reduced activity of basic fibroblastic growth factor in endothelial cells (85). AGEs also alter the properties of the extracellular matrix. Thus, glycosylation of collagen type IV renders it resistant to degradation by MMP (86). AGE cross-linking of type I collagen expands the packing of collagen molecules (87). Glycosylation of a carboxy-terminal domain of type IV collagen interferes with the normal assembly of collagen networks (88). AGE formation in laminin inhibits assembly of laminin polymers (89) and decreases binding to type IV collagen and heparan sulfate (79). Furthermore, AGE modification alters matrix-cell interactions. For example, modification of type IV collagen decreases endothelial cell adhesion (90). Last, AGE also may act through binding to receptors, the most well characterized being the receptor of AGE (RAGE) (81). It is interesting that stimulation of RAGE can lead to transdifferentiation of renal tubular epithelial cells to myofibroblasts (91), which may be responsible for accumulation of extracellular matrix.
The ability of RAGE signaling to cause diabetic complications was demonstrated directly in a study in which transgenic mice overexpressing iNOS targeted to insulin-producing cells, providing a model for type 1 diabetes, were cross-bred with transgenic mice overexpressing RAGE. Development of glomerular lesions was accelerated in these double-transgenic mice (92) and could be prevented by an AGE inhibitor (92).
AGE formation has effects on numerous vascular signaling molecules. Thus, they reduce the expression of eNOS (93) and increase the expression of ET-1 (94) in endothelial-cell culture. AGEs, through increased production of reactive oxygen species (ROS), activate NF-κB (95), which is responsible for induction of several growth factors. AGEs also increase expression of TGF-β in mesenteric vessels (96) and increase retinal VEGF expression (97). In endothelial-cell culture, AGEs, via RAGE, decrease production of prostacyclin, induce expression of plasminogen activator inhibitor-1 (PAI-1) (98), and induce expression of adhesion molecules in endothelium (99). A soluble receptor for RAGE inhibits barrier function in endothelial cells and vascular permeability in diabetic rats (100).
Many approaches to the pharmacologic inhibition of AGE formation are being attempted. Aminoguanidine, an inhibitor of AGE formation, has shown an effect on preventing nephropathy (101) and retinopathy (102) in animal models of diabetes. Newer agents are being developed, including 2,3-diaminophenazine, an inhibitor of AGE formation (103); ALT-711, which breaks AGE cross-links (104); pyridoxamine; and OBP-9195 (105). It is interesting that treatment with the ACE inhibitor ramipril decreased renal AGE accumulation to the same extent as did an inhibitor of AGE formation, aminoguanidine, perhaps by preventing activation of NAD(P)H oxidase [reduced form of nicotinamide adenine dinucleotide (phosphate)] (106). Results are not yet available from clinical trials with AGE inhibitors for the treatment of diabetic microvascular complications.
VASCULAR INFLAMMATION
Typical elements of inflammatory processes are evident in the vasculature in diabetes (107). Increased expression of vascular adhesion molecules leading to recruitment of leukocytes to the vascular wall is regarded as a pivotal event in early inflammation. Blocking intracellular adhesion molecule-1 (ICAM-1) prevents leukostasis and retinal vascular leakage. The pro-inflammatory cytokine tumor necrosis factor-α (TNF-α) is induced in rats with streptozotocin-induced diabetes (31).
Treatment of such animals with high-dose aspirin; the cyclooxygenase-2 inhibitor meloxicam; or eternacept, which blocks TNF-α actions by competing with its tissue receptors, prevents increased ICAM-1 expression, leukostasis, capillary leakage, and upregulation of eNOS (31). Inflammation may therefore play a role in the development of microvascular complications of diabetes, but current information on this subject is sparse.
Treatment of such animals with high-dose aspirin; the cyclooxygenase-2 inhibitor meloxicam; or eternacept, which blocks TNF-α actions by competing with its tissue receptors, prevents increased ICAM-1 expression, leukostasis, capillary leakage, and upregulation of eNOS (31). Inflammation may therefore play a role in the development of microvascular complications of diabetes, but current information on this subject is sparse.
Intracellular Consequences of Altered Metabolism
INCREASED INTRACELLULAR GLUCOSE CONCENTRATIONS
Interventional trials of hypoglycemic drug therapy have unequivocally linked hyperglycemia with the development and progression of microvascular complications (108,109,110). Lowering blood glucose levels has been less successful in preventing atherothrombotic complications, suggesting that factors other than hyperglycemia may be more important for the development of macrovascular complications. The perception of hyperglycemia as the primary cause for microvascular complications explains why the vast majority of studies of the mechanisms responsible have focused on the effects of increased glucose concentrations, even though multiple other metabolic alterations exist in diabetes.
Many of the mechanisms on the cellular level described below involve alteration of cellular homeostasis caused by increased cellular glucose uptake and increased intracellular glucose concentration. This is prevented in part in tissues where insulin resistance decreases cellular glucose uptake. Insulin resistance can thus be viewed as a compensatory mechanism to avoid excessive intracellular glucose concentrations; this has prompted the speculation that tissues that do not have this capability are those prone to diabetic complications (111). Aortic endothelial and smooth muscle cells express GLUT1 (a glucose transport protein not responsive to insulin) but do not express GLUT2 to GLUT5 (112). VSMC can downregulate GLUT1 in response to increasing extracellular glucose concentrations, but endothelial cells cannot (112), thus making endothelial cells more susceptible to the effects of hyperglycemia. Similarly, overexpression of GLUT1, which is not regulated by insulin, in mesangial cells cultured in normal glucose concentrations increased collagen metabolism and accumulation (113).
Characterization of the regulation of glucose transporters in different tissues prone to microvascular complications is not complete, and the dogma given above may not be valid in all tissues. For example, GLUT1, the primary glucose transporter in the retina, is downregulated in diabetes (114). Likewise, insulin stimulates glucose uptake and glycogen synthesis in retinal capillary endothelial cells, but—as expected from the expression of GLUT isoforms—not in aortic endothelial cells (115).
INTRACELLULAR REDOX STATUS AND OXIDATIVE STRESS
Oxidative stress, i.e., an imbalance between ROS and cellular antioxidant defense systems (116,117), may result from alterations of glucose metabolism or be secondary to activation or dysregulation of several enzymes not directly involved in glucose metabolism. In population-based cohorts, vascular oxidative stress, measured by plasma markers, is associated with diabetes, even after adjustments are made for several covariables (118). Furthermore, patients with diabetes have a depletion of the reduced form of intracellular glutathione (119), but an increase in plasma extracellular superoxide dismutase, which is associated with severity of retinopathy and nephropathy (120). Oxidative stress has been demonstrated in organs affected by complications of diabetes; thus, in the vitreous body of the eye of patients with diabetes, lower levels of oxidative metabolites are associated with better blood glucose control (121). On the other hand, intervention against oxidative stress has been shown to prevent organ damage in animal models. For example, transgenic mice overexpressing superoxide dismutase are protected from renal complications of streptozotocin-induced diabetes (122), and long-term treatment of diabetic rodents with antioxidants has had beneficial effects on the development of complications in the retina (123), the kidney (124), and the peripheral nerve (125,126).
In patients with diabetes, smaller trials have shown that dietary supplementation with vitamin E lowers the albumin excretion rate (127,128) and creatinine clearance (128), increases retinal blood flow (128), and improves nerve conduction (129). However, in a substudy of HOPE (Heart Outcomes Prevention Evaluation), currently the largest completed clinical trial of antioxidant therapy, vitamin E supplementation did not decrease the need for retinal laser therapy or improve the urine albumin-creatinine ratio (130).
Oxidative stress may cause cellular dysfunction by promoting formation of AGEs, by inducing DNA strand breaks and activating poly(ADP-ribose) polymerase (PARP) (131,132), by causing dysfunction of eNOS, and by activating p38 and other stress-activated pathways leading to apoptosis. These and other mechanisms are discussed below.
Polyol Pathway
Increased cellular glucose uptake increases the flux of glucose through the polyol pathway (also known as the sorbitol pathway), which consumes NADPH by the aldose reductase reaction and reduces NAD+ (the oxidized form of nicotinamide adenine dinucleotide) by the sorbitol reductase reaction (133). Activation of the polyol pathway not only may result from increased availability of glucose, the upstream substrate for the pathway, but also may be secondary to inactivation of GAPDH, thus diverting glucose from glycolysis to other pathways of glucose metabolism (132). An overactive polyol pathway will therefore deplete cytosolic NADPH, which is necessary to maintain the primary intracellular antioxidant, glutathione, in its reduced state. Aldose reductase inhibition reduces neuropathy in diabetic dogs (134) and patients with diabetes (135), but although early studies in animal models of diabetes showed promise with regard to an effect on retinopathy or nephropathy, such effects have not been demonstrated in patients with diabetes (134,136).
Pentose Phosphate Pathway
Superoxide Production from Mitochondria
In mitochondria, the citric acid cycle provides NADH and FADH2(reduced form of flavin adenine dinucleotide), which can act as electron donors for the electron transport chain, creating a proton gradient over the inner mitochondrial membrane. If intracellular glucose concentrations are increased, yielding excessive reducing equivalents for this process, the proton gradient will be high and inhibit the transfer of electrons from reduced coenzyme Q (ubiquinone) to complex III of the electron transport chain (140). Instead, electrons will be transferred to molecular oxygen, producing superoxide.
Vascular NAD(P)H Oxidase
The most important source of superoxide quantitatively in the vascular wall is thought to be an NAD(P)H oxidase that resembles the phagocytic NADPH oxidase but favors NADH as a substrate (141). This oxidase is expressed in endothelial cells (142) and VSMCs (141). Expression and activity of vascular NAD(P)H oxidase is increased in rat models of type 1 (143) and type 2 (144) diabetes. This enzyme may be activated by an increase in the NADH/NAD+ ratio, which in diabetes may be caused by an increased flux through the polyol pathway (see above) or activation of PARP (131). The lactate/pyruvate concentration ratio is an index of cytosolic
NADH/NAD+ ratio, as pyruvate reduces NAD+ in the lactate dehydrogenase reaction. In endothelial-cell cultures, superoxide production is increased by the addition of lactate and decreased by the addition of pyruvate (145). Apart from regulation by cofactor requirements, vascular NAD(P)H oxidase may be regulated by alterations in signaling pathways. Thus, NAD(P)H oxidase activity may be increased by elevated concentrations of glucose and free fatty acids through PKC activation (146), and its expression may be increased by ET-1 (147).
NADH/NAD+ ratio, as pyruvate reduces NAD+ in the lactate dehydrogenase reaction. In endothelial-cell cultures, superoxide production is increased by the addition of lactate and decreased by the addition of pyruvate (145). Apart from regulation by cofactor requirements, vascular NAD(P)H oxidase may be regulated by alterations in signaling pathways. Thus, NAD(P)H oxidase activity may be increased by elevated concentrations of glucose and free fatty acids through PKC activation (146), and its expression may be increased by ET-1 (147).
eNOS Dysfunction
Oxidative stress may inhibit NO-mediated endothelial function by degrading NO (148). Alternatively, it may lead to decreased intracellular concentrations of the reduced, active form of BH4, a cofactor for eNOS. The reason for this may be that BH4 either cannot be kept in the reduced form because of decreased NAPDH concentrations (139) or because BH4 is oxidized, preferentially by reaction with peroxynitrite (149). Suboptimal BH4 concentrations lead to “uncoupling” of the oxidase and reductase domain of NO synthase (150), which results in the oxidation of molecular oxygen instead of L-arginine and the synthesis of superoxide instead of NO. Thus, BH4 deficiency may be both cause and effect of vascular oxidative stress (151). Uncoupling of eNOS by peroxynitrate may also be caused by disruption of a zinc-thiolate cluster that stabilizes eNOS as a dimer (152).
In studies of fructose-fed rats, a model of insulin resistance, endothelial BH4 concentrations were reduced and concentrations of oxidized BH4 were increased (153,154). Furthermore, superoxide generation was increased, NO production was decreased, and endothelium-dependent vasorelaxation was impaired. The functional abnormalities were improved by ex vivo exposure of vascular tissue to BH4 (153). Oral administration of BH4 increased endothelial BH4 concentrations and improved the functional abnormalities (154). In patients with type 2 diabetes, intrabrachial infusion of BH4 increased forearm endothelium-dependent vasodilation (155).
Tyrosine Nitration
Superoxide reacts with NO with a very high rate constant. The product is the highly reactive intermediate peroxynitrate, which may react with a host of intracellular molecules. One such target is the tyrosine residues of proteins, a reaction that may result in tyrosine nitration (29). Mg-superoxide dismutase (SOD) seems to be a particularly susceptible target for such modification, which decreases its catalytic activity, thus potentially further aggravating oxidative stress (156). Examples of other enzymes modified in this manner include certain metabolic enzymes (157) and prostacyclin synthase (158).
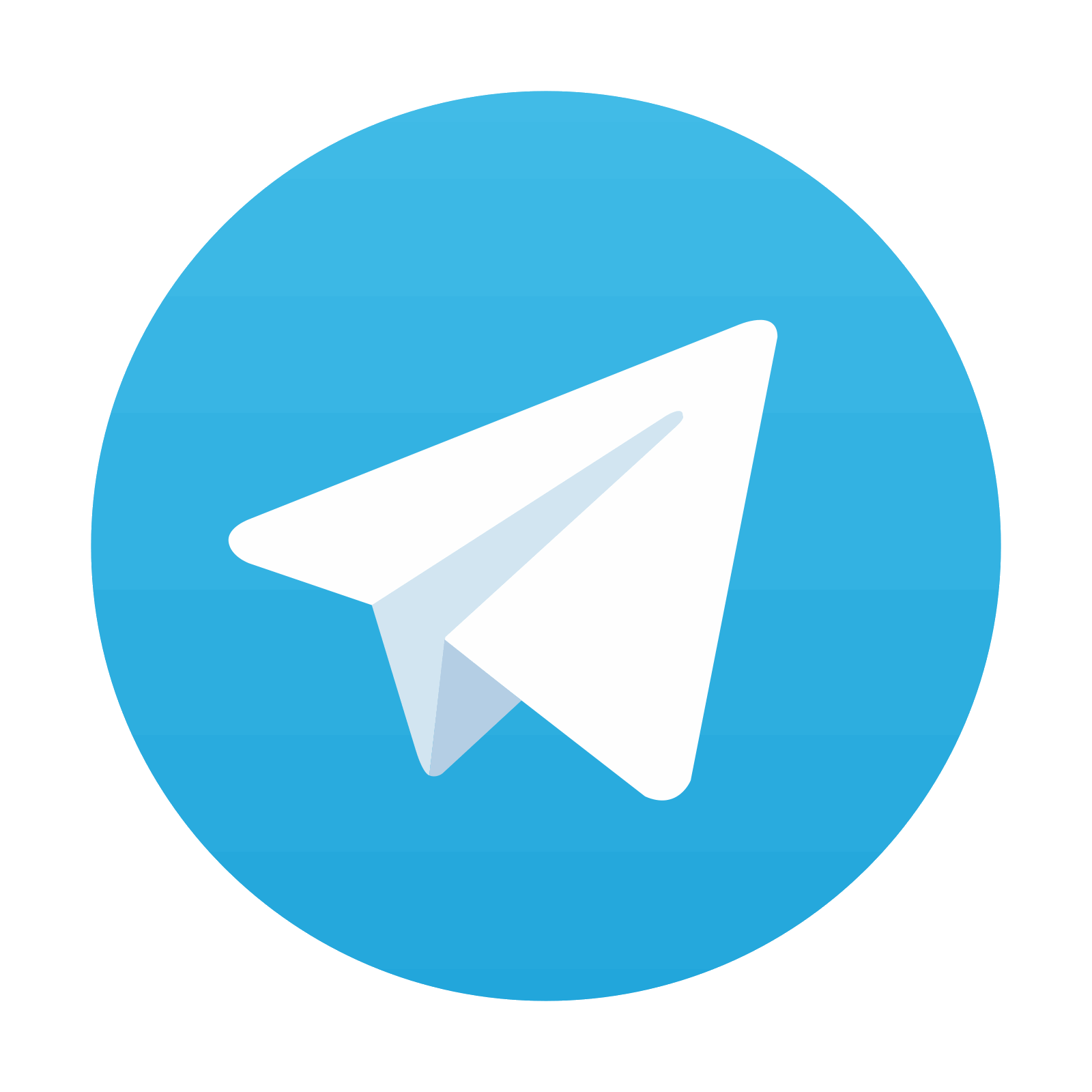
Stay updated, free articles. Join our Telegram channel
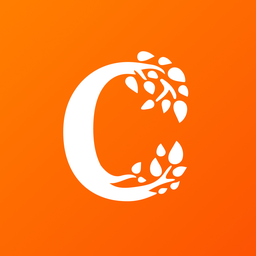
Full access? Get Clinical Tree
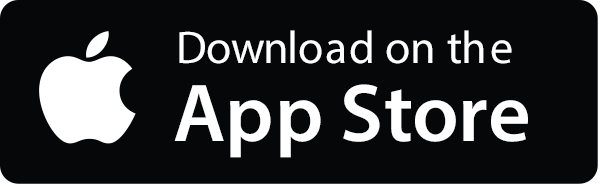
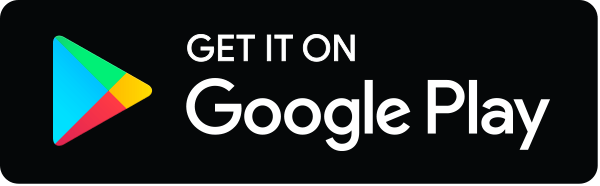
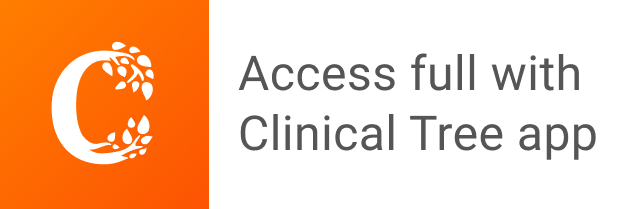