Chapter 9 Iron deficiency anaemia and iron overload
Iron metabolism
The iron content of the body and its distribution among the various proteins is summarized in Table 9.1. Most of the iron is present in the oxygen-carrying protein of the red blood cell, haemoglobin, the synthesis and breakdown of which dominates iron turnover. Haem of haemoglobin is synthesized in nucleated red cells in the bone marrow by a pathway ending with the incorporation of iron into protoporphyrin IX by ferrochelatase. Haem breakdown from haemoglobin takes place mainly in phagocytic cells, largely those in the spleen, liver and bone marrow. Iron is released from haem by haem oxygenase and is largely reused for haem synthesis. Every day about 30 mg iron is used to make new haemoglobin and most of this is obtained from the breakdown of old red cells.
Table 9.1 Distribution of iron in the body in a 70 kg mana
Protein | Location | Iron content (mg) |
---|---|---|
Haemoglobin | Red blood cells | 3000 |
Myoglobin | Muscle | 400 |
Cytochromes and iron sulphur proteins | All tissues | 50 |
Transferrin | Plasma and extravascular fluid | 5 |
Ferritin and haemosiderin | Liver, spleen and bone marrow | 100–1000 |
a The numbers are different for women.
The iron-binding protein transferrin is responsible for extracellular transport. Each molecule of transferrin can bind up to two molecules of ferric iron. Most cells obtain iron from diferric transferrin which binds to transferrin receptors (TfR) on the cell surface. This is followed by internalization into vesicles, release of iron, transport of iron into the cytoplasm and recycling of the apotransferrin (the protein without iron) into the plasma (Fig. 9.1).
Dietary Iron Absorption
Iron absorption1 depends on the amount of iron in the diet, its bioavailability and the body’s need for iron. A normal Western diet provides approximately 15 mg iron daily. Of that iron, digestion within the gut lumen releases about half in a soluble form, from which only about 1 mg (5–10% of dietary iron) is transferred to the portal blood in a healthy adult male.
Regulation of Iron Absorption
Iron absorption may be regulated both at the stage of mucosal uptake and at the stage of transfer to the blood. Recent studies show that in mice HIF-2α, a mediator of cellular adaptation to hypoxia, regulates DMT1 transcription and thus mucosal uptake of iron.2 As the epithelial cells develop in the crypts of Lieberkuhn, their iron status reflects that of the plasma (transferrin saturation) and this programmes the cells to absorb iron appropriately as they differentiate along the villus. Transfer to the plasma depends on the requirements of the erythron for iron and the level of iron stores. This regulation is mediated directly by hepcidin, a peptide synthesized in the liver in response to iron and inflammation.3
Cellular Iron Uptake and Release
Diferric transferrin binds with high affinity to the transferrin receptor (TfR1) on the membrane of the cell to form a complex which initiates clathrin-dependent endocytosis of the local membrane. The resulting endosome contains the holotransferrin–transferrin receptor complex.4 The pH of the endosome is reduced by a proton pump and a conformational change in holotransferrin is induced causing iron release. Iron is then reduced by a membrane-bound ferrireductase (six transmembrane epithelial antigen of the prostate 3, STEAP3 in erythroid cells5) and transported into the cytoplasm by DMT1. This iron is then either stored as ferritin or used within the cell. Iron destined for haem and mitochondrial iron sulphur (Fe-S) clusters is transported into mitochondria by mitoferrin for incorporation into protoporphyrin by ferrochelatase6 and for Fe-S cluster assembly on the scaffold protein ISCU.7 Export by ABCB7 of an as-yet unknown component to the cytoplasm stimulates Fe-S cluster protein assembly there, essential for cellular iron homeostasis.7 Apotransferrin and the transferrin receptor of the endosome return rapidly to the cell surface where they dissociate at neutral pH so that the cycle can start again.7a
Hepcidin synthesis in the hepatocyte is controlled at the transcriptional level through the alteration of levels within the nucleus of different transcription factors able to bind to the gene. Interaction of diferric transferrin, bone morphogenetic proteins (BMPs), interleukin (IL)-6 and other inflammatory cytokines with cell surface receptors TfR1, TfR2, hemojuvelin (HJV) and IL-6 receptor lead to upregulation of the hepcidin gene through different and sometimes interacting signalling pathways. TMPRSS6, a plasma membrane serine protease, inhibits the HJV-BMP-SMAD signaling pathway by cleaving HJV from the surface of the cell, thus preventing overproduction of hepcidin and maintaining iron homeostasis.8,9 A soluble form of HJV (sHJV) produced within the cell by the enzyme furin and released into the plasma antagonizes and also inhibits this pathway.10
The mechanism of action of the HFE protein is less clear. HFE binds to both TfR1 and TfR2, decreasing the affinity of each for transferrin. Stabilization and endocytosis of TfR2 stimulates hepcidin production and there is evidence that diferric transferrin displaces the protein HFE from TfR1, leaving it free to interact with TfR2, thus stimulating hepcidin production in response to plasma iron levels.11–13
Increased erythropoiesis causes decreased hepcidin, increased iron absorption and increased iron availability for haemoglobin production. So far, two plasma antagonists of the BMP-HJV-SMAD pathway, GDF15 and TWSG1, produced by erythroid cells and associated with expanded ineffective erythropoiesis, have been found.14,15 Alteration of the function of key regulators leads to body iron overload or iron deficiency (see later). The extent and the manner in which these regulatory pathways vary between different tissues await further investigation.
Iron Storage
All cells require iron for the synthesis of proteins but have the ability also to store excess iron. There are two forms of storage iron: a soluble form, known as ferritin and insoluble haemosiderin.16 Ferritin consists of a spherical protein (molecular mass 480 000) enclosing a core of ferric-hydroxy-phosphate, which may contain up to 4000 atoms of iron. Haemosiderin is a denatured form of ferritin in which the protein shells have partly degraded, allowing the iron cores to aggregate. Haemosiderin deposits are readily visualized with the aid of the light microscope as areas of Prussian-blue positivity after staining of tissue sections with potassium ferrocyanide in acid (see Chapter 4). Ferritin is found in all cells and in the highest concentration in liver, spleen and bone marrow.
Regulation of Iron Metabolism
The expression of a number of iron proteins involved in both transport and storage is largely controlled posttranscriptionally by iron regulatory proteins (IRP1 and 2).4 The conformation of IRP1 required for binding to mRNA iron-responsive elements (IREs) and the turnover of IRP2 are directly affected by the amount of iron within a cell. Depending on where the target IRE elements are found these proteins may inhibit or enhance translation of many proteins involved in iron metabolism.
Plasma Iron Transport
Almost all the iron in plasma is tightly bound to transferrin, which is usually less than 50% saturated with iron. Transferrin, when incompletely saturated with iron, exists in four forms: apo, monoferric (iron bound at the ‘A’ site), monoferric (B) and diferric. The distribution may be determined by urea-polyacrylamide electrophoresis.17 Delivery to cells requires specific binding to transferrin receptors. The plasma iron pool (transferrin-bound iron) is about 3 mg, although the daily turnover is more than 10 times his amount. In addition, smaller amounts of iron are carried in the plasma by other proteins.
Haptoglobin is a serum glycoprotein that avidly binds haemoglobin αβ dimers released into the bloodstream by haemolysis. The haemoglobin–haptoglobin complex is rapidly removed from the plasma by a specific receptor, CD163, highly expressed on tissue macrophages.18
Hemopexin19 is a plasma glycoprotein of molecular mass approximately 60 kDa that binds haem and transports the haem to cells by a process that involves receptor-mediated endocytosis and recycling of the intact protein.
Low concentrations of ferritin are found in the plasma and ferritin concentrations in healthy subjects reflect body iron stores. Much of this ferritin appears to be glycosylated and has a relatively low iron content.20 Such ferritin has a half life (T½) of approximately 30 h. Ferritin is also released into the circulation as a result of tissue damage (most strikingly after necrosis of the liver). Tissue ferritin is cleared rapidly from the circulation (T½ in approximately 10 min) by the liver.
Non-transferrin-bound iron describes a form of iron that is not bound to transferrin, is of low molecular mass and can be bound by specific iron chelators.21 Several assays have been described that have demonstrated such a fraction in plasma from patients with iron overload. The chemical form of this iron is unknown, but it is probably rapidly removed from the circulation by the liver. Recent studies implicate the involvement of the zinc transporter ZIP14 in this process.22
Iron status
Normal iron status implies a level of erythropoiesis that is not limited by the supply of iron and the presence of a small reserve of ‘storage iron’ to cope with normal physiological needs. The ability to survive the acute loss of blood (iron) that may result from injury is also an advantage. The limits of normality are difficult to define and some argue that physiological normality is the presence of only a minimal amount of storage iron23 but the extremes (i.e. iron deficiency anaemia and haemochromatosis) are well understood.23a
Apart from too little or too much iron in the body, there is also the possibility of a maldistribution (Fig. 9.2). An example is anaemia associated with inflammation or infection where there is a partial failure of erythropoiesis and of iron release from the phagocytic cells in liver, spleen and bone marrow, which results in accumulation of iron as ferritin and haemosiderin in these cells. Determination of iron status requires an estimate of the amount of haemoglobin iron (usually by measuring the haemoglobin concentration (Hb) in the blood; see Chapter 3) and the level of storage iron (measuring serum ferritin concentration). Iron deficiency should be suspected in hypochromic, microcytic anaemia, but in the early stages of iron deficiency red cells may be normocytic and normochromic. Another feature of iron deficiency is an increased concentration of protoporphyrin in the red cells; normally, there is a small amount present, but defective haem synthesis caused by lack of iron results in the accumulation of zinc protoporphyrin and occasionally free protoporphyrin may be increased as well.24
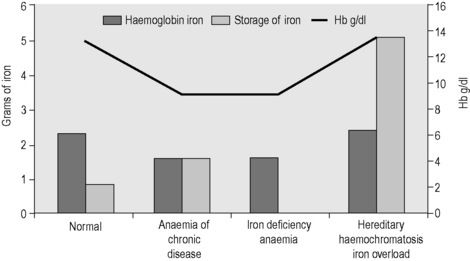
Figure 9.2 Body iron content and distribution in different conditions. Additional assays are sometimes required. In genetic haemochromatosis, early iron accumulation is indicated by increased transferrin saturation alone. The serum ferritin concentration increases only later as the level of stored iron increases. In the anaemia of chronic disease, patients often have normal serum ferritin concentrations even when storage iron in the bone marrow is absent (see p. 336). In this situation, the assay of serum transferrin receptor may help detect tissue iron deficiency.
Disorders of iron metabolism
Clinical aspects of iron metabolism have been reviewed25–27 and are summarized in Table 9.2. A guideline on the management of genetic haemochromatosis is available.28
Table 9.2 Disorders of iron metabolism
Iron deficiency |
Deficient iron intake |
Diet of low bioavailability |
Increased physiological requirements due to rapid growth in early childhood and in adolescence |
Blood loss: physiological (e.g. menstruation) or pathological (e.g. gastrointestinal) |
Malabsorption of iron intake |
Reduced gastric acid secretion (e.g. after partial gastrectomy) |
Reduced duodenal absorptive area (e.g. in coeliac disease) |
Rare, inherited iron-refractory iron deficiency anaemia |
TMPRSS6 deficiency |
Redistribution of iron |
Macrophage iron accumulation |
Inflammatory, infectious or malignant diseases (‘anaemia of chronic disease’) |
Iron overload |
Due to increased iron absorption |
e.g. Hereditary haemochromatosis – commonly homozygosity for HFE C282Y but sometimes involving other genes (HAMP,HJV,TFR2,SLC40A1) Substantial ineffective erythropoiesis (e.g. β thalassaemia syndromes of intermediate and major severity, some types of sideroblastic anaemia, congenital dyserythropoietic anaemia) Sub-Saharan iron overload (‘Bantu siderosis’) – only in combination with increased dietary iron Other rare inherited disorders (e.g. congenital atransferrinaemia, DMT1 deficiency) Inappropriate iron therapy (rare) |
Due to multiple blood transfusions in refractory anaemias |
e.g. Thalassaemia major |
Aplastic anaemia |
Myelodysplastic syndromes |
Methods for assessing iron status
The methods used to assess iron status are summarized in Table 9.3. Some are not generally applicable but have value in the standardization of indirect methods. The determinations of Hb and red cell indices are described in Chapter 3.
Serum Ferritin Assay
With the recognition that the small quantity of ferritin in human serum (15–300 μg/l in healthy men) reflects body iron stores, measurement of serum ferritin has been widely adopted as a test for iron deficiency and iron overload. The first reliable method to be introduced was an immunoradiometric assay in which excess radiolabelled antibody was reacted with ferritin and antibody not bound to ferritin was removed with an immunoadsorbent.31 This assay was supplanted by the two-site immunoradiometric assay, which is sensitive and convenient. Since then the principle of this assay has been extended to non-radioactive labelling, including enzymes (enzyme-linked immunosorbent assay or ELISA). Most current laboratory immunoassay systems for clinical laboratories include ferritin in the assay repertoire. Factors to be considered when selecting an immunoassay system are discussed later. The method described in the next section is an ELISA. The most sophisticated equipment required is a microtitre plate reader.
Immunoassay for Ferritin
Preparation and Storage of Ferritin
Ferritin may be prepared from iron-loaded human liver or spleen obtained at operation (spleen) or postmortem. The informed consent of the patient or the patient’s relatives should be obtained for use in preparation of ferritin. Tissue should be obtained as soon as possible after death and may be stored at −20°C for 1 year. The usual precautions must be taken to avoid the risk of infection when handling tissues and extracts. Ferritin is purified by methods that exploit its stability at 75°C. Further purification is obtained by precipitation from cadmium sulphate solution and gel-filtration chromatography.32 Purity should be assessed by polyacrylamide gel electrophoresis,33 and the protein content determined by the method of Lowry and colleagues, as described by Worwood.33 Buffered solutions of human ferritin may be stored at 4°C, at concentrations of 1–4 mg protein/ml, in the presence of sodium azide as a preservative, for up to 3 years. Such solutions should not be frozen. Ferritin, from human liver or spleen, may be obtained from several suppliers of laboratory reagents. This should be used as an assay standard only after calibration against the international standard (see p. 183).
Antibodies to Human Ferritin
High-affinity antibodies to human liver or spleen ferritin are suitable. Polyclonal antibodies may be raised in rabbits or sheep by conventional methods,34 and the titre checked by precipitation with human ferritin.33 An immunoglobulin G (IgG)-enriched fraction of antiserum is required for labelling with enzyme in the assay. The simplest method is to precipitate IgG with ammonium sulphate.35 Monoclonal antibodies that are specific for ‘L’ subunit-rich ferritin (liver or spleen ferritin) are also suitable. A mouse monoclonal antibody and a horseradish peroxidase (HRP)-labelled antibody are available from NIBSC (www.nibsc.ac.uk; codes 87/654 and 87/662). Suitable antibodies are available from commercial suppliers.
Conjugation of Antiferritin IgG Preparation to Horseradish Peroxidase36
Preparation and Storage of a Standard Ferritin Solution
Dilute a solution of human ferritin to approximately 200 μg/ml in water. Measure the protein concentration by the method of Lowry after diluting further to 20–50 μg/ml. Then dilute the ferritin solution (approx. 200 μg/ml) accurately to a concentration of 10 μg/ml in 0.05 mol/l sodium barbitone solution containing 0.1 mol/l NaCl, 0.02% NaN3 and BSA (5 g/l) and adjusted to pH 8.0 with HCl. Deliver 200 μl into 200 small polypropylene tubes, cap tightly and store at 4°C for up to 1 year. For use, dilute in Buffer B to 1000 ng/ml, then prepare a range of standard solutions between 0.2 and 25 ng/ml. Calibrate this working standard against the World Health Organization (WHO) standard for the assay of serum ferritin 94/572, recombinant human L type ferritin. (Information available at: www.nibsc.ac.uk.)