Hypoglycemia in the newborn and infant
Diva D. De León, MD, Paul S. Thornton, MD, BCh, MRCPI, Charles A. Stanley, MD and Mark A. Sperling, MD
PRINCIPLES OF GLUCOSE METABOLISM
PHYSIOLOGY OF PERINATAL GLUCOSE HOMEOSTASIS
HORMONAL AND METABOLIC SYSTEMS OF FASTING ADAPTATION
DEFINITION OF HYPOGLYCEMIA IN NEONATES AND INFANTS
CLINICAL SYMPTOMS AND SIGNS ASSOCIATED WITH HYPOGLYCEMIA
CLASSIFICATION OF CAUSES OF PERSISTENT HYPOGLYCEMIA IN THE NEONATE AND INFANT
Disorders of Insulin Excess or Actions
Defects in Counter-Regulatory Response
Defects in Glycogenolysis and Gluconeogenesis
Disorders of Fatty Acid Oxidation: Medium-Chain Acyl-Coenzyme A Dehydrogenase Deficiency (MCAD)
Principles of glucose metabolism
A systematic approach to hypoglycemia in the newborn, infant, or child requires an appreciation of the central role of glucose in the body’s fuel economy.1 Glucose metabolism accounts for approximately half of basal daily energy needs and is the principal metabolic fuel of the human brain. Glucose can be stored for energy in the form of glycogen and fat, and its carbon can be used for synthesis of protein and for structural components (such as cell membranes). The aerobic oxidation of glucose yields high energy by producing 36 mols of adenosine triphosphate (ATP) for each mol of glucose.
All glucose extracted by the brain is oxidized, and thus cerebral glucose utilization parallels cerebral oxygen uptake. In 5-week-old infants, cerebral glucose utilization already represents 71% to 93% of the adult level in most brain regions (ranging from 13 to 25 μmol/100 g/min). At that age, the areas with highest metabolic rates for glucose are the sensorimotor cortex, thalamus, midbrain, brainstem, and cerebellar vermis. By 3 months, metabolic rates for glucose increase in the parietal, temporal, and occipital cortices, as well as in basal ganglia. By 8 months, subsequent increases occur in the frontal cortex and various associative regions, concordant with the appearance of higher cortical and cognitive functions. Adult levels of cerebral glucose utilization (19 to 33 μmol/100 g/min) are reached by the time one is 2 years of age, and they continue to increase until 3 to 4 years of age—when they reach values ranging from 49 to 65 μmol/100 g/min, which are maintained to approximately 9 years of age. They then begin to decline, reaching adult levels by the end of the second decade.2
Glucose uptake by the brain occurs by means of a carrier-mediated facilitated diffusion process that is glucose concentration dependent, as well as energy, Na+, and insulin independent.3 This process is mediated by facilitative glucose transporter (GLUT) proteins. The human genome contains 14 members of the GLUT family. Each of these isoforms is expressed in a distinct tissue distribution. In most cells, GLUTs mediate the import of glucose, because hexokinase activity maintains low intracellular glucose concentrations. Characterization of the different members has provided new insights into the regulation and significance of glucose transport and its disorders in various tissues3,4,5 (Table 6-1). Several members of the GLUT family have been detected in the brain. GLUT1 is located at the blood-brain barrier,6 and although some neurons express GLUT2 and GLUT4, the majority use GLUT3 as their primary transporter.7 The main isoform present in insulin-responsive tissues is GLUT4.8,9
Of major importance is the confirmation at a molecular level of biochemical evidence that glucose entry into brain cells and its subsequent metabolism are not dependent on insulin but rather are dependent on circulating arterial glucose concentration.10 Therefore, a decrease in arterial glucose concentration or a defect in the glucose transport mechanism of the brain will result in intracerebral glucopenia and low cerebrospinal fluid glucose concentration (hypoglycorrhachia)—with attendant symptoms and signs of cerebral glucopenia as subsequently described.10–12
In normal humans, plasma glucose concentrations range from 3.9 to 7.1 mmol/L (70 to 128 mg/dL) and in the brain the range is from 0.8 to 2.3 mmol/L (14 to 41 mg/dL).13 Brain glucose consumption will outstrip glucose transport at plasma glucose concentration < 2 mmol/L (36 mg/dL) (brain glucose will approach 0 mmol/L).14 To prevent circulating arterial blood glucose from decreasing precipitously under normal physiologic conditions, and therefore to prevent impairment of vital function that depends on cerebral glucose metabolism, an elaborate defense mechanism has evolved.1 This defense against hypoglycemia is integrated by the autonomic nervous system and by hormones that act synergistically to enhance glucose production through enzymatic modulation of glycogenolysis and gluconeogenesis while simultaneously limiting peripheral glucose use.15,16 Thus, hypoglycemia is the result of a defect in one or several of the complex interactions that maintain a normal range of glucose concentration, preventing its fall to less than 3.9 mmol/L (70 mg/dL) during fasting and its rise to more than 7.8 mmol/L (140 mg/dL) during feeding.
These mechanisms are not fully developed at birth, when there is an abrupt transition from intrauterine life to extrauterine life. A neonate delivered prematurely whose enzymatic machinery mechanisms are not yet fully developed and expressed or one whose placental insufficiency resulted in intrauterine growth retardation with limited tissue nutrient deposits may be particularly vulnerable to hypoglycemia,17 often with consequences to subsequent cerebral development or function.18,19
Physiology of perinatal glucose homeostasis
Glucose metabolism in the fetus
It is estimated that 80% of fetal energy comes from glucose and that the remaining 20% is derived from metabolism of lactate and amino acids.20 Studies in human pregnancies as well as in other species suggest that fetal glucose is derived entirely from the mother through placenta transfer.21–24 Thus, maternal and fetal glucose concentrations behave as a single pool, with no endogenous glucose production in the fetus. There are important clinical consequences implicit in these findings. For example, acute hypoglycemia in a mother with diabetes will result in acute hypoglycemia in the fetus—with no ability to acutely compensate for the abrupt reduction in blood glucose supply.
The transfer of glucose across the placenta to the fetus is mediated by sodium-independent facilitated diffusion along a concentration gradient. This process is dependent on specific isoforms of the glucose transporters. GLUT1 has been identified as the major isoform in the human placenta,25 although other members of the family, such as GLUT3, are also expressed.26 Of note is the fact that the placental GLUTs are not regulated by insulin, suggesting that maternal glucose concentration and (more specifically) the maternal-fetal glucose gradient is the major determinant of placental glucose transfer independent of the maternal insulin concentration perfusing the placenta.27
The fetus, from early gestation to time of delivery, has only slightly lower concentrations of plasma glucose than maternal concentrations with a mean fetal glucose concentration of approximately 4.4 mmol/L (79 mg/dL). This maternal-fetal gradient allows greater transfer of glucose from the maternal circulation.28 There is a linear relationship between the maternal and fetal glucose concentration with glucose transported by facilitated carrier-mediated diffusion along the concentration gradient.29 As gestation proceeds this maternal-fetal gradient increases, perhaps reflecting the increased utilization of the fetal placenta unit.28 When maternal glucose rises > 20 mmol/L (360 mg/dL), glucose transport changes to simple diffusion. Of the maternal glucose taken up by the placenta, approximately 50% to 60% is used by the placenta and 40% to 50% transported to the fetus.30
In the second half of gestation, the fetal–placental unit secretes large quantities of human placental lactogen, progesterone, and estrogen, which cause increased maternal insulin resistance. Maternal insulin does not significantly cross the placenta unless it is bound to antibody.31 Fetal insulin secretion cannot rapidly respond to rapidly changing glucose levels. In utero, the raised insulin-to-glucagon ratio drives metabolism toward anabolism, as evidenced by the rapid rate of growth of the fetus. The insulin resistance of the mother results in an increased availability of glucose, amino acids, and lipids to meet the ever-increasing energy demands of the fetus, which is in a constant anabolic state with glucose utilized for both energy production and the formation of triglycerides stores and protein anabolism. Throughout gestation, the balance of glycogen metabolism is toward anabolism and the building of glycogen stores. As gestation advances, the fetal glycogen content in the liver increases to about 24.6 mg/g liver by 120 days. At 36 weeks, gestation there is a steep increase in accumulation of glycogen, with levels rising to 50 mg/g liver at term.
Changes at birth: Transition phase
The abrupt interruption of maternal glucose transfer to the fetus at delivery imposes an immediate need to mobilize endogenous glucose and to rapidly adjust insulin secretion to glucose concentration. Following birth and clamping of the cord, the newborn has to transition from a state of glucose excess and anabolism to a rapidly fluxing state of glucose deficiency and excess. This is due to the change from a constant supply of glucose and amino acids from the mother to variable and intermittent oral intake. In addition, in breast-fed infants the colostrum is low in carbohydrates and high in fat. This transition is regulated by the interplay of hormones and enzyme induction. At birth, plasma epinephrine and norepinephrine rise three- to tenfold,32 and may be responsible for changes in the insulin-to-glucagon ratio, which in utero is high and favors anabolism. Within the first 2 hours after birth, glucagon concentrations rise and continue to increase during the first 3 days of life; insulin, on the other hand, falls initially and remains in the basal range for several days.33 These changes induce both pyruvate carboxylase (PC) and phosphoenolpyruvate carboxykinase (PEPCK).34 By 8 to 12 hours, gluconeogenesis becomes fully effective.35 The ability to develop significant ketosis is impaired for the first 12 hours of life, as shown by a study in appropriate for gestational age (AGA) infants fasted up to 8 hours after birth. In these infants, free fatty acid (FFA) levels averaged 1.41 mmol/L (appropriately elevated) and total ketones were approximately 0.36 mmol/L (low relative to the FFA); thus, although insulin levels fall and lipolysis occurs, fatty acid oxidation with ketone generation does not occur.35 However, after 12 hours of life, ketone production and utilization occur at accelerated rates and equal those in adults starved for 48 to 72 hours.36 Thus, ketone utilization can provide up to 25% of the energy needs of the neonate after the first 12 hours of life.
With the rise in glucagon and epinephrine and the fall in insulin, glycogen is mobilized to produce glucose by activation of glycogen phosphorylase. Indeed, levels of glycogen decline from a peak of 50 mg/g liver just prior to birth, to very low concentrations (< 10 mg/g liver) in the first 24 hours of life, and glycogen contributes to almost 50% of the newborn’s glucose requirements during this time. Gluconeogenesis from pyruvate contributes approximately 20% to 30% of glucose needs and glycerol produced by lipolysis contributes approximately 20%.24,37
In summary, glucose concentration during intrauterine life is constant and > 3.9 mmol/L (70 mg/dL). Following birth, glucose concentration falls to a mean glucose of 3.1 mmol/L (56 mg/dL) at 2 hours (fifth percentile level 28 mg/dL), and as glycogenolysis and gluconeogenesis become established, glucose concentration rises to a mean of 3.5 mmol/L (63 mg/dL) (fifth percentile level 40 mg/dL) by 2 to 24 hours of life. By 24 hours of life, mean glucose concentrations are 3.8 mmol/L (68 mg/dL) (fifth percentile level 41 mg/dL) and by 48 hours they rise to a mean of 3.7 mmol/L (67 mg/dL) (fifth percentile level 48 mg/dL).38 As a result of these changes, 30% of normal newborns will have glucose concentrations < 2.8 mmol/L (50 mg/dL) in the first 24 hours of life and thereafter the frequency of glucose concentrations < 50 mg/dL in newborns older than 24 hours of age is 0.5%.17 This physiologic drop in glucose levels in the first 24 hours of life is transitional hypoglycemia, which by definition occurs in normal healthy newborns. It should be differentiated from both transient and persistent pathologic causes of hypoglycemia.
Abnormalities of transition
In infants born IUGR, the diminished glycogen reserves may have a significant impact on glucose control in the first 24 hours of life, resulting in hypoglycemia greater than expected. However, there are data that also suggest that small for gestational age (SGA) infants have a transient state of hyperinsulinism persisting over the first days of life as they adjust to extrauterine life.39 Premature infants may also not have accumulated adequate glycogen and will have immaturity of their enzyme systems also increasing the risk of hypoglycemia. Infants of diabetic mothers or infants with perinatal stress hyperinsulinism, or even those with genetic defects in insulin secretion or other conditions, will not have the sudden drop in insulin levels after birth, thus impairing glycogenolysis and lipolysis and increasing the risk of hypoglycemia. It is important for the clinician to recognize these at-risk neonates and neonates whose glucose control is out of the range of transitional hypoglycemia during this period and treat appropriately. In particular, it is important to recognize conditions in which insulin secretion is increased, as these infants will neither mobilize glycogen nor be able to oxidize fatty acids, thus putting their brains at risk of damage due to the combination of hypoglycemia and hypoketosis.
Management of hypoglycemia in the first 24 hours
Management of hypoglycemia in the first 24 hours of life is important from two points of view. First, the management plan should avoid treatment of infants who do not need treatment; second, it should include a management plan for those at risk of having a permanent hypoglycemic disorder that both identifies those neonates and threats them adequately. It is not currently recommended that all newborns have glucose concentration measured. However, if an infant has symptoms consistent with hypoglycemia—such as lethargy, apnea, or seizures, or is unwell or in the at-risk category (e.g., infants with siblings with known hypoglycemic disorders, preterm infants, LGA infants, SGA infants, infants of a diabetic mother, or infants who had birth asphyxia)—the infant’s glucose levels should be measured.
If the baby has symptoms of hypoglycemia or is unwell and the glucose is < 2.8 mmol/L (50 mg/dL), then IV glucose should be administered to keep the glucose > 3.3 mmol/L (60 mg/dL).40 Typically doses of glucose would be a 200 mg/kg (2 mL/kg dextrose 10%) bolus dose followed by a starting glucose infusion rate (GIR) of 4 to 6 mg/kg/min (60 to 90 mL dextrose 10% /kg/day) and rising by GIR of 1.6 mg/kg/min (1 mL/kg dextrose 10%/hour) until glucose levels are > 3.3 mmol/L (60 mg/dL) are obtained. Glucose levels should be checked every 15 minutes and the glucose infusion rate increased until the desired range is achieved. If after two dose increases of 1.6 mg/kg/min (1 mL/kg dextrose 10%/hour) the glucose levels remain < 3.3 mmol/L (60 mg/dL), then an increase by 3.2 mg/kg/min (2 mL/kg dextrose 10%/hour) every 15 minutes is recommended.
There is no evidence-based medicine to show us how to differentiate neonates who failed the preceding protocols because of a more severe transition from neonates with a more serious and potentially harmful cause of hypoglycemia. Both the American Academy of Pediatrics guidelines41 and the Canadian Pediatric Society guidelines40 recommend further testing to identify neonates who may needing longer-term treatment and to identify when glucose metabolism has returned to normal, but they do not recommend how and when this should be done.
Hormonal and metabolic systems of fasting adaptation
Three metabolic systems regulate the physiologic response to fasting: (1) hepatic glycogenolysis, (2) hepatic gluconeogenesis, and (3) hepatic ketogenesis. The key enzymatic steps in these pathways are shown in Figure 6-1. These metabolic systems are coordinated by the endocrine system, consisting of suppression of insulin (the most important endocrine response to fasting, as insulin suppresses all three metabolic systems) balanced by the secretion of glucagon, epinephrine, cortisol, and growth hormone. Table 6-2 summarizes the counter-balancing effects of these counter-regulatory hormones on key metabolic pathways. There is a hierarchic redundancy in the interaction of these counter-regulatory hormones that provides a margin of safety (“fail-safe mechanism”) if only one counter-regulatory hormone is impaired. Epinephrine and glucagon are quick acting, each signaling its effects by activation of cyclic AMP. Deficiencies of glucagon, as occurs in long-standing type 1 diabetes mellitus, can be largely compensated for by an intact autonomic nervous system with appropriate α- and β-adrenergic, and cholinergic effects. Conversely, autonomic failure can be largely compensated for if glucagon secretion remains intact.1
FIGURE 6-1 Key metabolic pathways of intermediary metabolism. Disruption of the elements of these pathways may be pathogenetic in the development of hypoglycemia. Not shown is the hormonal control of these pathways. Indicated are (1) glucose 6-phosphatase, (2) glucokinase, (3) phosphorylase, (4) phosphoglucomutase, (5) glycogen synthetase, (6) phosphofructokinase, (7) fructose 1,6-diphosphatase, (8) fructose 1,6-diphosphate aldolase, (9) phosphoenolpyruvate carboxykinase, and (10) pyruvate carboxylase. (From Pagliara AS, Karl IE, Haymond M, Kipnis DM [1973]. Hypoglycemia in infancy and childhood. J Pediatr 82:365-379 and 82:558-577.)
Hepatic glycogenolysis is sufficient to meet energy requirements for only a few hours. Beyond that time, glucose must be produced by hepatic gluconeogenesis from precursors such as amino acids, glycerol, and lactate recycled from glycolysis. The major source of gluconeogenic precursors is muscle protein. Although the pool of muscle protein is large, it is required for body function and thus in contrast to stores of glycogen and fat there are no “reserves” of protein to draw on during fasting. To spare the use of essential protein during extended fasting, glucose consumption must be suppressed by switching on the mobilization and oxidation of fatty acids from adipose triglyceride stores, producing ketone bodies and glycerol. The ketone bodies inhibit phosphofructokinase, hexokinase, and inactivate pyruvate dehydrogenase, thus reducing glucose utilization in the liver and preserving glucose for those cells dependent of glucose alone.
The essential function of fasting adaptation is to maintain fuel supply to the brain. Glucose homeostasis is very limited in neonates and infants compared to adults, in part because of their smaller reserves of liver glycogen and muscle protein but also because of their relatively larger rates of glucose consumption due to their larger brain-to-body-mass ratio. For example, the fuel stores of a 10-kg infant are only 15% of those of an adult. However, the caloric needs are 60% of those of an adult and glucose turnover rates per kilogram are two- to threefold greater. As shown in Figure 6-2, early in fasting, glucose is the primary brain fuel and accounts for over 90% of total body oxygen consumption. Glucose is provided chiefly from hepatic glycogenolysis, supplemented by hepatic gluconeogenesis utilizing amino acids released by muscle protein turnover. After 8 to 12 hours in normal infants (24 to 36 hours in adults), glucose production declines, because the supply of liver glycogen is limited and the rate of gluconeogenesis from amino acids remains constant. At this time, a transition to fat as the major fuel for the body begins, with accelerated adipose tissue lipolysis and increased fatty acid oxidation in muscle and ketogenesis in liver. Lipolysis also generates glycerol, which is an important gluconeogenic substrate once fasting adaptation is fully active. The brain cannot utilize fatty acids directly, because they do not pass the blood-brain barrier. However, the brain can substitute glucose consumption with the ketones acetoacetate and β-hydroxybutyrate, which are released by the liver as the end product of hepatic fatty acid oxidation. In late stages of fasting adaptation, fatty acid oxidation and ketone utilization account for 90% of total oxygen consumption.
FIGURE 6-2 Contribution of major fasting systems to brain metabolism over time in a typical normal infant. Note that glycogen stores are depleted by 8 to 12 hours and that ketogenesis becomes the major source of brain substrate by 24 hours.
The operation of the metabolic and endocrine systems of fasting adaptation is evidenced by the changes in circulating levels of metabolic fuels and hormones during a fast. As shown in Figure 6-3, in young infants a 24-hour fast is accompanied by a gradual fall in plasma glucose levels as hepatic glycogen stores are depleted, a progressive fall in concentrations of gluconeogenic substrate (e.g., lactate, alanine) as they are used for hepatic gluconeogenesis, a brisk rise in free fatty acids as lipolysis is activated, and a dramatic rise in β-hydroxybutyrate (the major ketone) as hepatic ketogenesis is turned on.1,42
FIGURE 6-3 In infants, a 24-hour fast is accompanied by a gradual fall in plasma glucose levels as hepatic glycogen stores are depleted, a progressive fall in concentrations of gluconeogenic substrate (e.g., lactate, alanine) as they are used for hepatic gluconeogenesis, a brisk rise in free fatty acids (FFA) as lipolysis is activated, and a dramatic rise in β-hydroxybutyrate (BOB) (the major ketone) as hepatic ketogenesis is turned on.
By the time that plasma glucose concentration has fallen to < 2.8 mmol/L (50 mg/dL), all the metabolic systems and the hormonal responses described earlier will have fully engaged. A “critical sample” drawn at this time will therefore enable an evaluation of these systems and detect abnormalities of fasting adaptation. As an example, when insulin concentration is above the lower limit of detection according to the assay at the time when plasma glucose concentration is 2.8 mmol/L (50 mg/dL) or less, there is a hyperinsulinemic state reflecting failure of the mechanisms that normally result in suppression of insulin secretion during fasting or hypoglycemia.43–45 Thus, a snapshot of the integrity of the metabolic and endocrine fasting systems can easily be obtained by measuring the plasma levels of the major fuels and hormones at the end of a fast, when the blood glucose approaches hypoglycemic levels (“critical samples”) (Table 6-3; also see also Box 6-2, presented later in the chapter). The use of these critical samples in diagnosing the cause of hypoglycemia is discussed later.
Definition of hypoglycemia in neonates and infants
Beyond the first 48 hours of life, normal plasma glucose concentration in neonates and infants are not different than in older children and adults. Thus, plasma glucose concentrations in the postabsorptive state range between 3.9 and 5.6 mmol/L (70 and 100 mg/dL), with a mean of 4.4 to 4.7 mmol/L (80 to 85 mg/dL). A plasma glucose level of 2.8 mmol/L (50 mg/dL) is conventionally used as an end point for provocative diagnostic tests for hypoglycemia. This value is low enough to strongly stimulate the endocrine and metabolic defenses against hypoglycemia for identifying the mechanism responsible for hypoglycemia. Falling glucose levels elicit a typical sequence of responses: plasma insulin levels begin to decrease when plasma glucose falls to the range of 4.4 to 4.7 mmol/L (80 to 85 mg/dL) and insulin secretion is generally “switched off” at glucose concentrations below 2.5 to 3 mmol/L (45 to 54 mg/dL); glucagon secretion increases when plasma glucose levels are in the range of 3.6 to 3.9 mmol/L (65 to 70 mg/dL); epinephrine, cortisol, and growth hormone responses are activated in the range of 3.6 to 3.9 mmol/L (65 to 70 mg/dL). As the plasma glucose falls below 3.3 mmol/L (59 mg/dL), auditory and visual reaction time is prolonged and cognitive function begins to decline as the plasma glucose concentration declines below 2.5 to 3.5 mmol/L (45 to 63 mg/dL), with some of this variability dependent of the testing employed.13,46
A number of potential artifacts can interfere with measuring glucose levels in neonates and infants (Box 6-1). Whole-blood glucose concentrations are 10% to 15% lower than plasma glucose levels because erythrocytes have a higher concentration of protein (hemoglobin) versus plasma with its higher water content, and hence higher dissolved glucose concentrations. The difference may be greater in neonates with higher hematocrits. Blood samples that are not processed promptly can have erroneously low glucose levels, owing to glycolysis by red and white blood cells. At room temperature, the decline of whole-blood glucose can be 5 to 7 mg/dL/hr. The use of inhibitors, such as fluoride, in collection tubes avoids this problem.
For infants beyond the transitional period, treatment should be initiated promptly for plasma glucose values below 2.8 mmol/L (50 mg/dL). Plasma glucose values below 3.3 mmol/L (60 mg/dL) should be rechecked, and treatment considered if values are confirmed to be below 3.3 mmol/L (60 mg/dL). Symptomatic infants and neonates should be treated with intravenous dextrose (0.2 g/kg bolus, followed by infusion at 5 to 10 mg/kg/min). These rates approximate the normal hepatic glucose production rates in neonates and young infants (Figure 6-4). Asymptomatic neonates with plasma glucose levels below 2.8 mmol/L (50 mg/dL) may be treated with oral glucose, but only if there is good reason to believe the problem is a transient one that will not recur. This essentially only applies to otherwise normal neonates during the first 12 to 24 hours after birth who have delayed feedings, such as with initiation of breastfeeding. Beyond the first day of life, all neonates with verified plasma glucose concentration less than 2.8 mmol/L (50 mg/dL) should be suspected of having a hypoglycemic disorder.
FIGURE 6-4 Glucose production versus body weight determined in 19 newborns with the use of stable isotopic techniques. These studies provide support for the calculated rates of glucose administration required to correct hypoglycemia. (From Bier DM, Leake RD, Haymond MW, et al. [1977]. Measurement of “true” glucose production rates in infancy and childhood with 6,6-dideuteroglucose. Diabetes 26:1016.)
Clinical symptoms and signs associated with hypoglycemia
The clinical features of hypoglycemia in infants may be associated with both neurogenic and neuroglycopenic components (Table 6-4). Symptoms are often subtle and not specific, therefore a high index of clinical suspicion must be maintained. Any alteration in clinical status in a newborn that suggests a change in neurologic behavior, fall in temperature, change in feeding pattern, or presence of tremors must be considered a possible initial presentation of a hypoglycemic episode. A seizure must always be considered a possible manifestation of hypoglycemia.
Diagnostic approach
Important facts from the history include the duration of fasting that provoked hypoglycemia. Onset within a few hours of a meal would be consistent with hyperinsulinism or glucose 6-phosphatase deficiency, whereas onset after 10 to 12 hours would be consistent with a defect in fatty acid oxidation. Pituitary deficiency with growth hormone or adrenocorticotropic hormone (ACTH)-cortisol deficiency might be suspected by the presence of midline facial malformations, microphthalmia, or microphallus (follicle-stimulating hormone [FSH]/luteinizing hormone [LH] deficiency in utero). Growth failure is also a prominent feature of glucose 6-phosphatase deficiency or debrancher deficiency glycogen storage disease after the first 3 months of life. Both of these disorders are associated with massive hepatomegaly. Abnormal results of liver function tests (transaminases) and hyperammonemia, with or without elevated creatine kinase level, would suggest a possible fatty acid oxidation disorder (see Table 6-3).
Figure 6-5 outlines an algorithm for diagnosis of different forms of hypoglycemia based on readily available laboratory tests on the “critical” blood and urine samples. The first discriminant is a measure of acidemia at the time of hypoglycemia using the serum bicarbonate. If the acidemia is caused by elevations of the ketoacids (β-hydroxybutyrate and acetoacetate), possibilities include a normal child fasted for too long (ketotic hypoglycemia), a defect in glycogenolysis (glycogen storage disease type 3), or counter-regulatory hormone deficiency (hypopituitarism). If the acidemia is caused by an elevation of lactic acid, a block of gluconeogenesis should be suspected (glucose 6-phosphatase or fructose 1,6-diphosphatase deficiency or ethanol ingestion).
FIGURE 6-5 Algorithm for diagnosis of hypoglycemia based on “critical” blood tests obtained during a period of hypoglycemia. FFA, free fatty acids; FAO: fatty acid oxidation; GSD, glycogen storage disorder; SGA, small for gestational age. (Modified from Stanley CA, Baker L [1978]. Hypoglycemia. In Kaye R, Oski FA, Barness LA (eds.), Core textbook of pediatrics. Philadelphia: JB Lippincott, 280-305.)
If there is no acidemia (i.e., absence of the normal elevation of ketones) but free fatty acid levels are high, a defect in fatty acid oxidation and ketogenesis should be suspected (MCAD deficiency). If ketones are not appropriately increased but the free fatty acid concentrations are also suppressed, hyperinsulinism should be suspected. In the neonatal period, the features of hyperinsulinism can be mimicked by congenital pituitary deficiency. Further tests can then be planned using the initial critical specimens to confirm the suspected diagnosis. These may include physiologic tests (such as the glucagon stimulation test at a time of hypoglycemia to confirm hyperinsulinism) or specialized laboratory tests (such as a plasma acyl-carnitine profile) to identify a defect in mitochondrial β-oxidation.
Classification of causes of persistent hypoglycemia in the neonate and infant (box 6-2)
Disorders of insulin excess or actions
Suppression of insulin secretion is critical for maintaining euglycemia during fasting through the activation of hepatic glycogenolysis, gluconeogenesis, and fatty acid oxidation. Indeed, failure to suppress insulin secretion or actions is the most common cause of persistent hypoglycemia in neonates and children. Whether this is the result of a genetic defect in factors involved in the insulin secretory pathway or in the insulin signaling cascade, the clinical and biochemical hallmarks in the neonate are: (1) increased birth weight because of the growth-promoting effects of insulin in utero, (2) increased glucose utilization, (3) suppression of lipolysis and ketogenesis (low free fatty acids and ketones at the time of hypoglycemia), and (4) inappropriate glycemic response to glucagon at time of hypoglycemia (an increase of > 30 mg/dL) (Box 6-3). These features are critical for making the diagnosis, but it is also essential to determine the underlying cause because the treatment and prognosis may vary among the different disorders. The potential risk of brain damage in an infant suffering from hyperinsulinism is high, placing this diagnosis in a critical position in the diagnostic evaluation of neonatal hypoglycemia.
Hyperinsulinemic hypoglycemia
Transient hyperinsulinism resulting from maternal factors
Transient hyperinsulinism is a well-recognized complication in neonates after a pregnancy complicated by gestational diabetes. At birth, infants born to these mothers may be large and plethoric—and their body stores of glycogen, protein, and fat are replete. The classic clinical description of the effect of hyperinsulinism relates to the infant of the diabetic mother47:
Prolonged neonatal hyperinsulinism: Perinatal stress-induced hyperinsulinism
As shown in Figure 6-6, the risk of postnatal hypoglycemia is increased in neonates who are small for gestational age. There is increasing evidence that prolonged hypoglycemia in some neonates exposed to perinatal stress such as birth asphyxia, maternal toxemia, prematurity, or intrauterine growth retardation or other peripartum stress is due to hyperinsulinism.48–50 The estimated incidence of prolonged neonatal hyperinsulinism is 1:12,000 live births.50
FIGURE 6-6 Incidence of plasma glucose level being less than 30 mg/dL before first feeding at 3 to 6 hours of age in newborns, classified by birth weight and gestational age. (From Lubchenko LO, Bard H [1971]. Incidence of hypoglycemia in newborn infants by birth weight and gestational age. Pediatrics 47:831.)
The clinical presentation of perinatal stress-induced hyperinsulinism is characterized by high glucose utilization, and the response to fasting hypoglycemia shows an elevated plasma insulin level (although it may be normal), low β-hydroxybutyrate and free fatty acid levels, and inappropriate glycemic response to glucagon at the time of hypoglycemia. Unlike the transient hyperinsulinism seen in the infant of the diabetic mother, perinatal stress-induced hyperinsulinism may persist for several days to several weeks. In a series of neonates with perinatal stress-induced hyperinsulinism, the median age of resolution was 6 months.50 The mechanism responsible for the dysregulated insulin secretion is not known. Acute insulin responses (AIRs) show that in general the patterns of insulin response to secretagogues (calcium, tolbutamide, glucose, and leucine) in infants with prolonged neonatal hyperinsulinism resembled those of normal controls.50
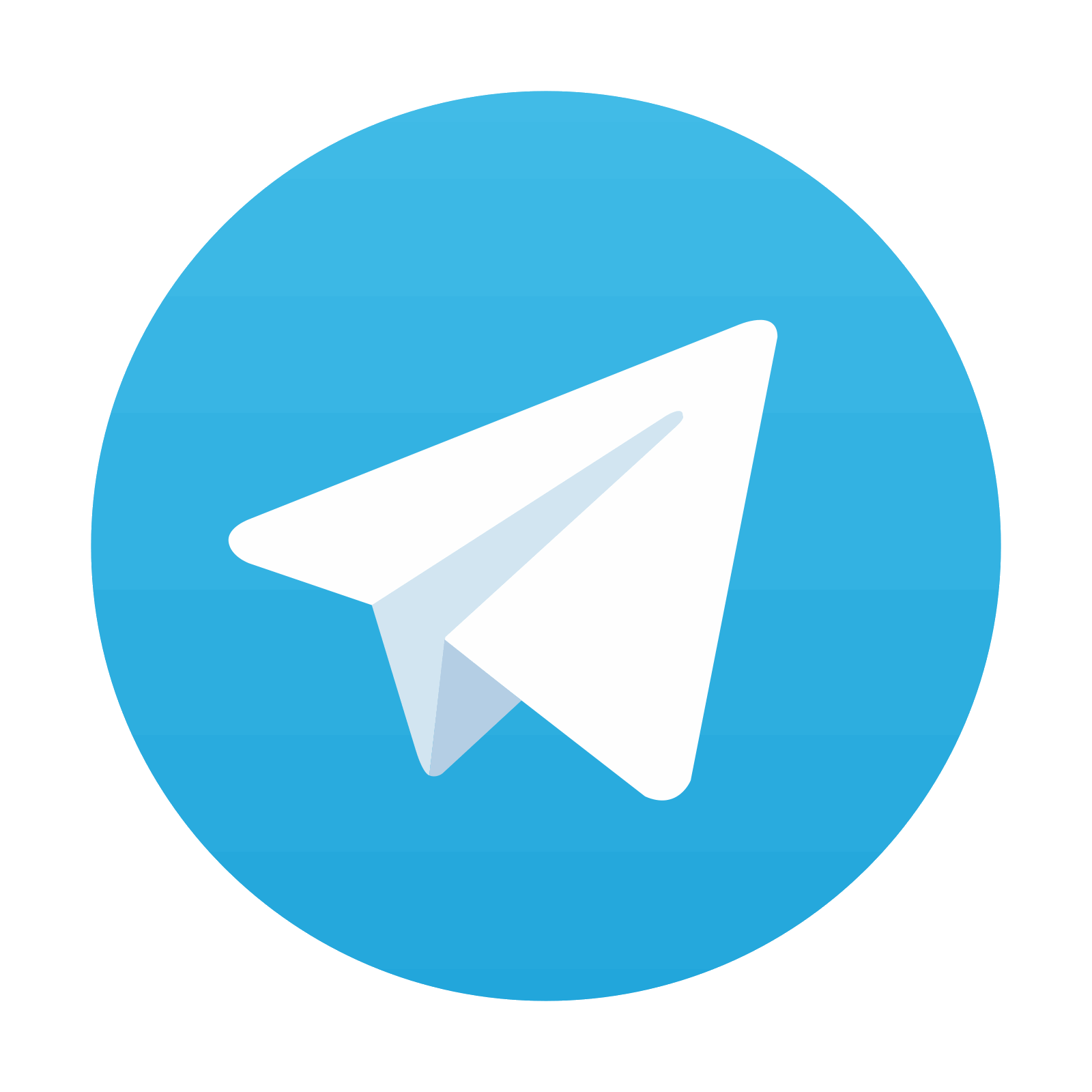
Stay updated, free articles. Join our Telegram channel
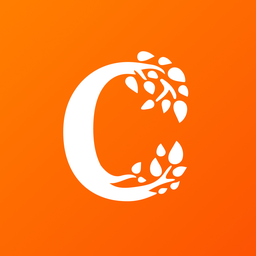
Full access? Get Clinical Tree
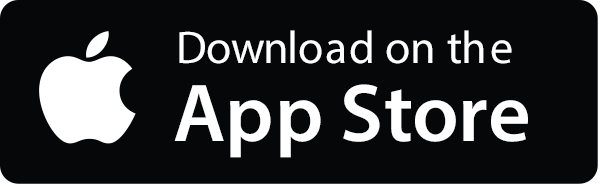
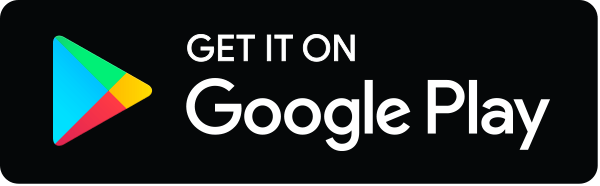