Robert L. Penn
Francisella tularensis (Tularemia)
Francisella tularensis is a gram-negative pathogen primarily of animals and occasionally of humans. The disease it causes is now recognized as tularemia in most areas of the world, but it has been called rabbit fever, deer fly fever, and market men’s disease in the United States; wild hare disease (yato-byo) and Ohara’s disease in Japan; and water-rat trappers’ disease in Russia. Tularemia continues to be responsible for significant morbidity and mortality, despite the availability of numerous antibiotics active against the organism.1
F. tularensis infections have become a public health issue, with increasing concerns regarding military or terrorist uses of the organism in biological warfare. Because of this, tularemia was returned to the list of reportable diseases in the United States in 2000, after being excluded in 1995, and F. tularensis has once again become the subject of intense investigation. With heightened surveillance has come an appreciation of the continued occurrence, often in outbreaks, of natural F. tularensis infections throughout the world.
History
Tularemia has been so intimately linked to investigators in the United States that it has been referred to as an “American achievement.”2 However, its history includes important contributions from many other areas of the world, including Japan and the former Soviet Union. Hare-associated illness compatible with tularemia has been known in Japan since 1818, and perhaps the earliest written description of a patient with unmistakable tularemia was provided by Soken Honma in 1837.3
Credit for identifying the organism and recognizing the important clinical syndromes belongs to U.S. workers. In 1911, while evaluating possible plague outbreaks after the San Francisco earthquake of 1906, McCoy described a plaguelike illness common in the California ground squirrel, and with Chapin he successfully cultured the causative agent in 1912.4 They named it Bacterium tularense because this work took place in Tulare county. The first human case to have bacteriologic confirmation was an ocular infection reported in 1914.5,6 Although the cause was unknown at the time, tularemia was transmitted by contact with biting flies in Utah and was termed deer fly fever. Dr. Edward Francis, working for the U.S. Public Health Service, established the true cause of deer fly fever as B. tularense (a full decade after the organism was discovered in squirrels), proved the deer fly was the vector, and named the human disease tularemia to emphasize the frequent accompanying bacteremia. Francis also contributed to work that improved methods for cultivating B. tularense and making a serologic diagnosis, identified tick and other reservoirs for its transmission, clarified the clinical syndromes associated with tularemia, and emphasized the risk to laboratory workers and consumers from infected sources. For this lifetime of achievements, the genus in which the organism is classified was renamed Francisella in his honor.
In Japan, Ohara had described a rabbit-associated febrile disease, transmitted the illness to his wife by rubbing rabbit hearts over her hand, and recovered an organism from her lymph nodes; Francis later showed that this Japanese organism was identical to B. tularense. Tularemia was recognized in Astrakhan, Russia, in 1926, and scattered serious outbreaks occurred throughout the country during the subsequent decades. Scientists in the former Soviet Union also have intensively studied the disease and its causative organism.
Microbiology
Francisella organisms are small, aerobic, catalase-positive, pleomorphic, gram-negative coccobacilli. They are more uniformly rod-shaped during logarithmic growth, during which they tend to exhibit bipolar staining with Gram or Giemsa methods; this staining pattern accentuates a coccoidal appearance. The cell wall of F. tularensis has an unusually high level of fatty acids that have a profile unique to the genus, and wild strains possess an electron-transparent lipid-rich capsule. Loss of the capsule may lead to loss of serum resistance and virulence but may not diminish viability or survival within neutrophils7; however, the capsule is neither toxic nor immunogenic.
Francisella spp. belong to the Gammaproteobacteria and may be categorized on the basis of growth characteristics, biochemical reactions, and virulence properties (Table 229-1). The family Francisellaceae includes three species in the genus Francisella and four subspecies of F. tularensis.8–10 Although all have been associated with human disease, only the tularensis and holarctica subspecies of F. tularensis are relatively common. F. tularensis subsp. tularensis, also referred to as type A, is found almost exclusively in North America and is the most virulent species. Although previously thought to be restricted to North America, F. tularensis subsp. tularensis has been isolated in Europe and its identity verified using molecular techniques.11 F. tularensis subsp. holarctica, also referred to as type B, is found predominantly in Asia and Europe but also in North America; it is less virulent in humans and of low virulence in rabbits. The F. tularensis live vaccine strains are derived from F. tularensis subsp. holarctica (see later under “Vaccination”). Francisella novicida was previously classified as a separate species, but it is now known to be a subspecies of F. tularensis.8 F. tularensis subsp. novicida is of low virulence. Strains isolated from a restricted area in central Asia have been designated F. tularensis subsp. mediasiatica, are of low virulence, and produce acid from glycerol but not glucose.9 Strains isolated in Japan have been designated F. tularensis subsp. holarctica biovar japonica, but their differentiation from other F. tularensis subsp. holarctica strains on the basis of traditional phenotypic testing was not possible.10
TABLE 229-1
Characterization of Francisella Species
FEATURE | F. TULARENSIS SUBSPECIES* | F. PHILOMIRAGIA | F. HISPANIENSIS | ||
tularensis | holarctica | novicida | |||
Cysteine growth requirement | + | + | – | – | – |
Growth in broth plus 6% NaCl | – | – | +† | +‡ | NA |
Motility | – | – | – | – | NA |
Oxidase | – | – | – | +‡ | + |
Nitrate reduction | – | – | – | – | NA |
Acid from: | |||||
Glucose | +† | +† | +† | +† | + |
Glycerol | + | – | + | + | + |
Gelatin hydrolysis | – | – | – | † | NA |
Relative virulence | |||||
Humans | High | Intermediate | Low | Low | Low |
Rabbits | High | Low | Low | NA | NA |
* The fourth F. tularensis subspecies, F. tularensis subsp. mediasiatica, and the japonica biovar of F. tularensis subsp. holarctica are described in the text.
† Variable or delayed.
‡ Using Kovacs test; negative using cytochrome-oxidase test.
NA, not available; NaCl, sodium chloride.
Francisella philomiragia was previously called Yersinia philomiragia. It was reclassified because it shares the unique fatty acid profile of the Francisella and substantial DNA relatedness to this genus, although it has some unique biochemical features (see Table 229-1) and DNA hybridization patterns that distinguish it from F. tularensis. F. philomiragia is of low virulence for humans, although it has been isolated from muskrats and a dog.9,12 It is naturally found in brackish and salt water, and in liquid media it may form biofilms and interact with free-living amebas.13 All strains originally tested produced β-lactamase and were most susceptible to aminoglycosides, cefoxitin, cefotaxime, fluoroquinolones, tetracycline, and chloramphenicol. However, an infection caused by F. philomiragia resistant to cefazolin and cefotaxime has been reported.14 The most active agents against six strains in a standardized broth microdilution assay were the aminoglycosides and the fluoroquinolones.15 The complete genome of F. philomiragia has been sequenced, and it encodes a class A carbapenemase.16,17
Francisella hispaniensis was isolated from the blood and urine of a septic patient in Spain 1 month after undergoing lithotripsy.18 The organism was recently classified as a separate species of Francisella based on its phenotypic and genotypic properties (see Table 229-1).8
The taxonomy of Francisella has been complicated because biochemical reactions may be variable, weak, or delayed and also in part because of the different terms given to organisms isolated in different areas of the world. Classification of Francisella has been advanced by the sequencing of the whole genome from representative strains of F. tularensis subsp. tularensis (strain Schu S4), F. tularensis subsp. holarctica (including the attenuated live vaccine strain), and F. tularensis subsp. novicida, along with application of various molecular typing methods.11,19–22 These have included 16S ribosomal DNA gene sequence analysis, microarray analysis of the whole genome, multiple-locus variable-number tandem repeat analysis, canonical insertion-deletion markers, and single nucleotide polymorphism analysis.11,21 Reports using these techniques have supported the currently accepted taxonomy as outlined previously and in Table 229-1, demonstrated the utility of these methods for species and subspecies typing, and identified F. tularensis subsp. holarctica biovar japonica as a distinct group.22,23 Molecular typing has identified 3 genotypes of F. tularensis subsp. tularensis (designated A1a, A1b, and A2) and at least 10 genotypes of F. tularensis subsp. holarctica.24 Genomic analyses have indicated that there was a common F. tularensis ancestor for clonal subspecies evolution, that F. tularensis subsp. novicida is the oldest, and that F. tularensis subsp. tularensis appeared before F. tularensis subsp. holarctica, which is the youngest.25
F. tularensis requires cysteine or cystine (or another sulfhydryl source) for growth and therefore will not grow on most routine solid media or on gram-negative selective media such as MacConkey or eosin methylene blue agars. It may be recovered with the use of glucose cysteine blood agar, thioglycolate broth, chocolate agar suitable for gonococcal growth, modified Thayer-Martin medium, buffered charcoal-yeast agar, or cysteine heart agar with 9% chocolatized sheep blood.9,10 Blood agar may support growth of some F. tularensis isolates on initial plating but not on subpassage.26 Some strains of F. tularensis lack an overt requirement for cysteine or enriched medium for growth, and clinically significant strains of Francisella have been reported that do not show the expected fastidious growth characteristics.10 Francisella should be suspected, however, whenever a slowly growing, small, and poorly staining gram-negative coccobacillus is isolated on chocolate agar and grows poorly or not at all on blood agar. Visible colonies take 2 to 5 days to appear. Incubation at 35° C is optimal, with or without an atmosphere of increased CO2. The recovery of F. tularensis from contaminated specimens may be facilitated by plating them onto antibiotic-containing media known to support the growth of F. tularensis.9 Virtually all F. tularensis strains are positive for β-lactamase, and a weak class A β-lactamase native to F. tularensis has been identified.27
Differentiation of Francisella from other bacteria can be accomplished using direct fluorescent antibody staining, slide agglutination, polymerase chain reaction (PCR) assay, sequencing, or cellular fatty acid composition analysis.9 Antisera can distinguish between F. tularensis subsp. tularensis and F. tularensis subsp. novicida but not between F. tularensis subsp. tularensis and F. tularensis subsp. holarctica; strains within subspecies do not have antigenic differences detectable by antisera. It is important to note that automated laboratory identification systems should not be used for the identification of Francisella because they may generate aerosols and commonly misidentify F. tularensis as Haemophilus or Actinobacillus species.26 Whenever F. tularensis is suspected, the state public health laboratory should be notified immediately, and any local microbiologic testing should be done using a biological safety cabinet and following Biosafety Level 2 procedures. Isolates that cannot be excluded as F. tularensis should be sent to a reference laboratory in the Laboratory Response Network, typically the state public health laboratory. Federal regulations rigorously control possession and transport of F. tularensis and must be followed when sending an isolate to a referral laboratory for identification.
Virulence
F. tularensis produces no known exotoxins. The lipopolysaccharide (LPS) from the live vaccine strain of F. tularensis possesses at least 1000-fold less endotoxin activity than the LPS from Escherichia coli.28 This is in part because F. tularensis LPS has a unique structure and, unlike other gram-negative bacteria, it is not recognized by Toll-like receptor 4 (TLR4) and does not bind to LPS-binding protein.29 However, purified F. tularensis LPS can stimulate human B cells and, when present together with the F. tularensis heat shock protein GroEL, can synergistically activate human macrophages.30,31 The O-antigen side chains from F. tularensis subsp. tularensis and F. tularensis subsp. holarctica are identical but differ from that of F. tularensis subsp. novicida.29
Pili have been visualized on the surface of F. tularensis subsp. holarctica live vaccine strain and F. tularensis subsp. novicida; genome sequencing has detected genes homologous to those from other bacteria that encode type IV pili in F. tularensis subspp. tularensis, holarctica, and novicida; and type IV pili assembly contributes to virulence in murine models.32
Host immune responses are directed against numerous cell wall antigens, including membrane proteins, LPS, and carbohydrates, but previously it was not possible to identify dominant antigens. Proteomic analysis using serum from donors who have had tularemia or who have been vaccinated with the live vaccine strain has identified a large number of F. tularensis protein antigens and is able to identify among them possible immunodominant antigens.33 These have included many cytoplasmic and membrane proteins, as well as hypothetical proteins of unknown localization. Further proteomic analyses will be helpful to refine future diagnostic tests for tularemia and for the construction of effective vaccines.
Phenotypic correlates of virulence have included the capsule and citrulline ureidase activity. Wild encapsulated strains of F. tularensis are resistant to the bactericidal activity of normal serum, a capsule-deficient mutant of the live vaccine strain also is serum resistant, and the capsule-deficient mutant is less virulent in mice than its wild-type parent.34 The contribution of citrulline ureidase to virulence is unclear, and there are pathogenic isolates that do not possess this activity. Plasmids that have been found in isolates of F. tularensis subsp. holarctica, F. tularensis subsp. novicida, and F. philomiragia have not been found in the more virulent F. tularensis subsp. tularensis and thus are not essential for virulence. Several acid phosphatases (Acp) are present in Francisella, and they are important for its survival within macrophages. AcpA can inhibit the respiratory burst of neutrophils, expression of AcpA and histidine acid phosphatase (Hap) is induced by growth within macrophages, and AcpA is secreted into the cytosol of macrophages.35,36 Although deletion of acpA, acpB, acpC, and hap from F. tularensis subsp. novicida results in a mutant strain that is impaired in its ability to survive within macrophages and to escape from the phagosome, deletion of acpA and the combination of acpA, acpB, and acpC deletions do not alter the virulence of F. tularensis type A strain Schu S4.36,37 Mechanisms for iron acquisition are present in F. tularensis, and their disruption attenuates in vitro intracellular growth and organism virulence in a mouse model.38
Genomic and proteomic analyses have the potential to more specifically identify virulence factors in F. tularensis.22,39 F. tularensis contains a cluster of genes involved in virulence, the Francisella pathogenicity island (FPI). There are two copies of the FPI in F. tularensis subsp. tularensis and F. tularensis subsp. holarctica and one copy in F. tularensis subsp. novicida; although largely identical, the FPI from F. tularensis subsp. tularensis differs from that in the other subspecies.40,41 The FPI contains 19 genes required for murine virulence and intracellular growth in macrophages, including iglABCD and pdpABCD. FPI regulation involves at least six proteins that regulate many FPI genes, and some also regulate non-FPI genes.42,43 Intracellular growth, iron limitation, and oxidative stress also regulate expression of these genes.42,43 Disruption of many of these genes has been shown to impair the organism’s ability to survive within macrophages and significantly reduces virulence in animal models. Several proteins encoded by the FPI that have been linked to intracellular growth and virulence likely form part of a type VI secretion system.42,44,45 Many other genes can also be identified that probably play a role in Francisella virulence, and these are currently being explored.46
Other Francisella Species
A number of newly appreciated Francisella-like organisms have been identified by molecular testing or by culture.47 The same novel Francisella species was isolated from blood in a patient with pneumonia, and from cerebrospinal fluid in a different patient with meningitis.48 A survey of soil throughout Houston, Texas, using PCR amplification of DNA extracts identified multiple Francisella-like organisms that were distinct from known species of F. tularensis and F. philomiragia.49 Francisella-like organisms have been identified as a cause of granulomatous diseases in several fish species. They have been cultured, and 16S rRNA gene sequencing has shown them to be related most closely to F. philomiragia. However, they are currently believed to represent new Francisella species: F. noatunensis comb. nov. and F. noatunensis subsp. orientalis.50
Several endosymbiotic bacteria of ticks have been classified within the Francisellaceae family on the basis of 16S ribosomal gene sequence data. These include Wolbachia persica, an endosymbiont found in Rocky Mountain wood ticks termed Dermacentor andersoni symbiont, and symbiont B of Ornithodoros moubata.10 Similar organisms can be found in other hard and soft ticks, suggesting that they may be more widely distributed than previously observed. A Francisella-like organism has been found as an endosymbiont of a Paramecium species, and a related organism has been isolated from the waters off Hong Kong.51,52
Epidemiology
Distribution
Tularemia is widely distributed, but it is primarily a disease of the Northern Hemisphere and is most common between 30° and 71° north latitude. It has been remarkably absent from the United Kingdom, Africa, South America, and Australia. Whipp and co-workers53 reported the first case of tularemia from Australia in 2003, and it was caused by an F. tularensis subsp. novicida-like organism. Tularemia was very common in the United States before World War II. However, its incidence has declined steadily since the 1950s and has remained at fewer than 0.15 cases per 100,000 population since 1965.54,55 Because of its stable and low incidence, tularemia was removed from the list of nationally reportable diseases in 1995 but was added back in 2000 in part because of the concern about its use for bioterrorism. Since 2001, the case rates have been 0.05 per 100,000 population or less.55 Missouri, Arkansas, Oklahoma, Massachusetts, South Dakota, and Kansas reported 59% of the total U.S. cases from 2001 through 2010, with Dukes County in Massachusetts and Buffalo and Shannon Counties in South Dakota having the highest annual incidence rates (Fig. 229-1).54 In 2010, Arkansas, Missouri, Kansas, South Dakota, California, and Oklahoma reported 65% of the U.S. total number of tularemia cases.55 Groups with high incidence rates include Native Americans and Native Alaskans. Subtle changes have occurred in the geographic distribution of cases in the United States between 1965 and 1999.56 The southern border of tularemia has shifted northward so that fewer cases have been reported from south central states in recent years, and this is consistent with the predicted effects of climate change on tularemia’s geographic distribution.56 Environmental data also have been used to develop a model to predict risk for tularemia in specific geographic regions.57 More detailed investigation using molecular typing has found that specific F. tularensis strains may be geographically limited and that strain variation is related to virulence and disease severity.24 F. tularensis subsp. tularensis group A1 was found primarily in the central United States, including the states reporting the highest numbers of tularemia cases, and also in California; F. tularensis subsp. tularensis group A2 was found primarily in the western United States at higher elevations than the A1 isolates.58 The distribution of group A1 isolates correlated with the distribution of Amblyomma americanum and Dermacentor variabilis ticks and the eastern cottontail rabbit (Sylvilagus floridanus); the distribution of group A2 isolates correlated with the distribution of D. andersoni and the deer fly Chrysops discalis and also with the mountain cottontail rabbit (Sylvilagus nuttalii). There were only a few isolates of F. tularensis subsp. novicida, but most were found in the southeastern United States.58 In contrast, isolates of F. tularensis subsp. holarctica were widely dispersed geographically.58 Testing of additional U.S. isolates has identified two A1 genotypes, A1a and A1b. Genotype A1b isolates were significantly more likely to be from invasive infections and were associated with significantly higher mortality in humans than A1a and A2 isolates.59 Additional instances of the geographic restriction of specific F. tularensis strains have been identified as newer molecular typing methods have been more widely applied.24,60
Incidence
Historically, in the United States tularemia incidence has been most frequent in June through August and in December. The summer peak corresponds to a greater number of tick-acquired cases, whereas the smaller peak in winter reflects an increased number of hunting-associated cases. However, only the peak in the late spring and summer was prominent in more recent years. A review of 316 available F. tularensis human isolates from 39 states collected between 1964 and 2004 found that 208 (66%) were F. tularensis subsp. tularensis and 108 (34%) were F. tularensis subsp. holarctica.61 Most isolates of both subspecies occurred between May and September; a very small increase in numbers in December was noted only for F. tularensis subsp. tularensis and not for F. tularensis subsp. holarctica, and only F. tularensis subsp. tularensis was associated with lagomorph exposure.61 Males account for the majority of cases, perhaps because of greater exposure opportunities, and tularemia can occur in individuals of any age (Fig. 229-2). In the United States, incidence rates are highest in children ages 5 to 9 years and in adult men older than 55 years (see Fig. 229-2).54,55 Occupations that have been associated with an increased risk for tularemia are laboratory worker, farmer, landscaper, veterinarian, sheep worker, hunter or trapper, and cook or meat handler.
Transmission
F. tularensis is capable of infecting hundreds of different vertebrates and invertebrates, but no more than a dozen mammalian species are important to its ecology in any geographic region. These include lagomorphs, particularly Sylvilagus and Lepus spp., and rodents such as voles, squirrels, muskrats, and beavers in North America; included in Eurasia are voles, hamsters, mice, and hares. Transmission of F. tularensis to humans occurs most often through the bite of an insect or contact with contaminated animal products. Other routes of transmission include aerosol droplets, contact with contaminated water or mud, and animal bites. Illness may occur in families or friends because of shared activities and exposures. Nonetheless, human-to-human spread does not occur.
Blood-feeding arthropods and flies are the most important vectors for tularemia in the United States. Ticks predominate in the Rocky Mountain states and eastward, whereas biting flies predominate in California, Nevada, and Utah. However, an increase in human tularemia noted in Wyoming between 2001 and 2003 was linked most often to transmission by biting flies and was associated with a simultaneous outbreak of tularemia in rabbits.62 In contrast, mosquitoes are the most frequent insect vector in Sweden and Finland, and they are also important in the former Soviet Union. At least 13 species of ticks have been found to be naturally infected with F. tularensis, and transovarial passage may occur. The dog tick (D. variabilis), wood tick (D. andersoni), and Lone Star tick (A. americanum) are commonly involved in North America. The organism may be present in tick saliva or feces and may be inoculated either directly or indirectly into the bite wound. Several outbreaks of tick-borne tularemia have involved F. tularensis subsp. holarctica, although this organism is more often linked to water, rodents, and aquatic animals; tick transmission traditionally has been associated with F. tularensis subsp. tularensis. Tularemia in children in endemic areas of the United States is now most often associated with tick exposure in the summer.
Animal contact is another important mode of acquiring tularemia. Skinning, dressing, and eating infected animals, including rabbits, muskrats, beavers, squirrels, and birds, have transmitted tularemia, occasionally resulting in large outbreaks in hunters. Wild animals sold as pets are also potential vectors, as occurred in 2002 when infected prairie dogs were widely commercially distributed.63 The first outbreak in Spain was associated with processing hare carcasses and hare meat, and it was notable for a preponderance of women patients.64 Airborne transmission has occurred from these activities, as well as from contact with water, contaminated dust, and hay. An outbreak in 2000 of pneumonic tularemia on Martha’s Vineyard was associated with mowing lawns and using a brush cutter, and since then cases of pneumonic tularemia have been regularly encountered on Martha’s Vineyard each summer.65 Serologic evidence of F. tularensis infection among potential animal reservoirs on Martha’s Vineyard was found most frequently in raccoons (52.4%) and skunks (49.2%), although active infection in skunk and raccoon samples was not found by culture and PCR.66 Carnivorous animals may transiently carry F. tularensis in the mouth or on claws after killing or feeding on infected prey, whether or not they become infected. This is thought to be the mechanism by which domestic cats occasionally transmit tularemia, and in one case a buzzard.67
F. tularensis may survive for prolonged periods in water, mud, and animal carcasses even if frozen; however, cooking game meats thoroughly to the proper temperatures should minimize risk from ingestion. Nonetheless, contaminated food and water continue to be important environmental sources of tularemia, and disruptions of infrastructure caused by wars and natural disasters may be important contributing factors. In postwar Kosovo, an epizootic in increased rodent populations contaminated ransacked homes and food with F. tularensis, which led to foodborne and waterborne outbreaks among refugees returning to disrupted housing and sanitation.68,69 Although tularemia was not reported in Kosovo before these outbreaks, it has remained endemic since then and has spread throughout the country.68 The devastating effects of an earthquake in Turkey are believed to have resulted in water contamination and subsequent outbreaks of tularemia in the region.70 It has been demonstrated that F. tularensis subsp. tularensis, F. tularensis subsp. novicida, the live vaccine strain, and F. philomiragia will multiply intracellularly in Acanthamoeba castellanii and infect amebal cysts; F. tularensis subsp. tularensis is capable of surviving in amebal cysts for up to 21 days.13,71,72 Such a relationship may prove relevant to natural aquatic reservoirs for Francisella.
Pathogenesis
F. tularensis is a virulent organism for susceptible species, including the accidental human host. Although the organism is reported to penetrate intact skin, most investigators believe that penetration occurs through sites of inapparent skin disruption. The infectious dose in humans depends on the portal of entry: 10 to 50 organisms when injected intradermally or when inhaled, and 108 organisms when ingested. That low numbers of bacteria can cause infection through the skin, mucous membranes, and airways helps to explain in part the extreme risk that F. tularensis poses to laboratory workers. In general, F. tularensis subsp. tularensis causes more severe disease than either F. tularensis subsp. holarctica or F. tularensis subsp. novicida. The specific molecular reasons underlying these differences in virulence are unclear.
During the first 3 to 5 days after cutaneous inoculation, F. tularensis multiplies locally and produces a papule; ulceration may begin 2 to 4 days later.3 Organisms spread from the site of entry to regional lymph nodes and may disseminate via a lymphohematogenous route to involve multiple organs. Bacteremia is probably common in this early phase, although it is only occasionally detected. Changes in draining regional lymph nodes appear after skin lesions develop.3 An elegant description of the pathogenesis is offered by Geyer and colleagues.73 Infection with F. tularensis is characterized by an acute inflammatory response that involves fibrin, neutrophils, macrophages, and T lymphocytes. Neutrophils and macrophages surround earlier inflammatory cells, stimulated by the initial inoculum, that have become necrotic and degenerated. Eventually, lymphocytes, epithelioid cells, and giant cells migrate into the necrotic tissue. This extensive necrosis is noted in both lung tissue and lymph nodes. As the necrotic tissue expands, adjacent veins and arteries may thrombose. The organisms are usually present at the site of the necrotic tissue but are difficult to demonstrate on routine stains. Silver impregnation techniques (Steiner, Dieterle, and Warthin-Starry) enhance the visibility of the organisms, which are usually found in macrophages and epithelioid cells. Granulomas develop that occasionally may caseate; for this reason, specimens may be mistaken for tuberculosis. These changes can occur in any infected site and have been found at autopsy in lung, liver, spleen, lymph nodes, and bone marrow. Coalescence of necrotic foci may yield abscess formation. F. tularensis may remain viable in tissues for prolonged periods. The organism’s intracellular residence in the liver and other sites may help to protect it from host defenses and permit its early growth. F. tularensis is contained within granulomas in the livers of mice infected with the vaccine strain, and granuloma formation involves hepatic natural killer cells, interferon-γ (IFN- γ) production, and expression of inducible nitric oxide synthase.74
Most organisms recovered from blood in a mouse model of F. tularensis infection were free in plasma and not in leukocytes.75 The organisms grew well in whole blood but not in plasma, implying a requirement for host cells. These observations suggest that circulating F. tularensis may be taken up by leukocytes where they survive and are protected from innate humoral defenses and antibiotics, and then escape into the plasma where they can begin a cycle of reinfection. F. tularensis also can infect erythrocytes and persist within them, where they are protected from killing by gentamicin; this may perhaps contribute to relapse of tularemia after inadequate treatment.76
F. tularensis is a facultative intracellular parasite that is capable of growing within several different cell types, including macrophages, dendritic cells, hepatocytes, alveolar epithelial cells, and endothelial cells.77,78 However, macrophages are the primary site of its survival and replication. Entry into macrophages occurs through a unique mechanism involving engulfment by relatively capacious and asymmetrical pseudopod loops.77 Complement receptors, mannose receptors, class A scavenger receptors, Fcγ receptors, and surface nucleolin may be utilized for F. tularensis uptake, and the specific receptor pathway used may affect early suppression of innate defenses and intracellular trafficking of the organism.77,79,80 Inside the macrophage, virulent F. tularensis strains impair maturation of the endosome, thus avoiding phagosome-lysosome fusion. Phagosomes containing virulent F. tularensis are transiently acidified by the vacuolar adenosine triphosphatase pump, and organisms quickly escape into the cytosol. Bacteria proliferate in the cytoplasm, induce apoptotic cell death, and are released to further spread infection.81
Immunity
Humoral immunity, directed against carbohydrate antigens, develops between the second and third weeks after infection, with the almost simultaneous appearance of immunoglobulin M (IgM), IgG, and IgA agglutinating antibodies. However, antibodies alone are insufficient to protect against virulent F. tularensis infection.82 Opsonizing IgG and IgM antibodies are also produced, with the most efficient opsonization involving both immune serum and complement. Nonetheless, oxygen-dependent neutrophil killing of wild virulent strains is poor. Human neutrophils phagocytose opsonized F. tularensis organisms but do not kill them, and they escape into the neutrophil cytoplasm. Survival of F. tularensis within neutrophils is associated with inhibition of the reduced form of nicotinamide-adenine dinucleotide phosphate (NADPH) oxidase by multiple mechanisms, failure of the respiratory burst, and impaired neutrophil responsiveness to other activating stimuli.83 Neutrophil apoptosis is inhibited by F. tularensis, and survival of infected neutrophils is prolonged.84
Complete recovery from tularemia requires cell-mediated immunity, which is demonstrable approximately 1 week earlier than antibody responses and is directed against protein antigens. This cell-mediated immunity is α/β T-cell dependent but may involve either CD4+ or CD8+ T cells. Attempts are being made to define the critical molecular determinants that induce protective immunity. Current understanding about the immunopathogenesis of tularemia has been largely derived from mice infected with F. tularensis subsp. novicida or other strains less virulent for humans, and it is not known how this relates to human tularemia caused by more virulent organisms. Furthermore, different routes of infection and infection in different mouse strains may elicit different murine immune responses.85 IFN-γ and tumor necrosis factor-α (TNF-α) activate macrophages to kill F. tularensis through the production of nitric oxide and other reactive nitrogen products, although alveolar macrophages may use other mechanisms to inhibit the organism.86 Murine and human IFN-γ–activated macrophages also may inhibit growth of the virulent type A strain Schu S4 by mechanisms independent of reactive nitrogen and oxygen species.87
Several mechanisms are involved in the innate response that controls infection before the development of conventional cellular immunity. Early host defense against F. tularensis infection involves neutrophils, dendritic cells, macrophages, mast cells, TNF-α, IFN-γ, and interleukin-12, but these are not sufficient to resolve the infection. Neutrophils are less important in the lung than in systemic infection in mice, and neutrophils may contribute to lung damage.88 The initial response to F. tularensis infection is dependent on membrane-associated Toll-like receptor 2 recognition, particularly in the lung, and cytosolic nucleotide oligomerization domain–like receptors.85 In the mouse lung, virulent F. tularensis strains are capable of suppressing the expected early immune response.89,90 TLR4 recognition of F. tularensis LPS is poor; interestingly, resistance of mice to pulmonary infection with F. tularensis subsp. novicida is enhanced by pretreatment with a TLR4 agonist.91
For complete resolution of systemic infection, it is necessary that α/β+ T cells be functional and present after the initial defenses provided by the macrophages, dendritic cells, cytokines, and neutrophils. CD4+ and CD8+ cells each contribute to survival of mice infected with the vaccine strain or the virulent Schu S4 strain, and both are required to fully clear the infection.86,92 The contribution of α/β+ T cells involves TNF-α and IFN-γ production and is dependent on interleukin-12.85 In addition, it recently has been appreciated that both humoral and cellular immune mechanisms act together to achieve protective immunity.93,94 Natural infection or vaccination in humans results in long-lasting memory CD4+ and CD8+ cells.95 In mice, B cells contribute to protection against the attenuated and virulent strains that is not dependent on antibodies, but it is unknown if B cells play a similar role in human infection.86,92
F. tularensis is capable of suppressing or avoiding many aspects of humoral and cellular host defenses (see “Virulence”).96 It survives extracellularly in part because it is capable of avoiding complement-mediated lysis. It survives intracellularly by multiple mechanisms, including escape from the phagosome, suppression of the inflammasome, alternative activation of macrophages, and avoiding killing by cellular antimicrobial peptides.96 It also is capable of inhibiting IFN-γ signaling and production. For example, impaired clearance of F. tularensis live vaccine strain from mice with pulmonary infection may result from increased local prostaglandin E2 secretion and decreased numbers of IFN-γ–secreting T cells.97 Immunization of mice with F. tularensis may protect against subsequent challenge but not prevent persistent, low-level organ infection, and the essential determinants of sterilizing immunity remain unknown.92
Expansion of circulating γ/δ T cells has been documented in patients with acute tularemia. These cells respond to phosphoantigens from many different pathogens, including F. tularensis. The observed increase in the levels of Vγ9Vδ2 cells occurs after the first week of illness and may persist for longer than 1 year after infection.98 However, 10 to 30 years after natural infection, long-lived memory cells responsive to F. tularensis heat shock proteins are α/β T cells and not γ/δ T cells.99 Similarly, lymphocyte responsiveness to the live vaccine strain persists for up to 34 years after vaccination and IFN-γ expression involves both CD4+ and CD8+ cell populations.95
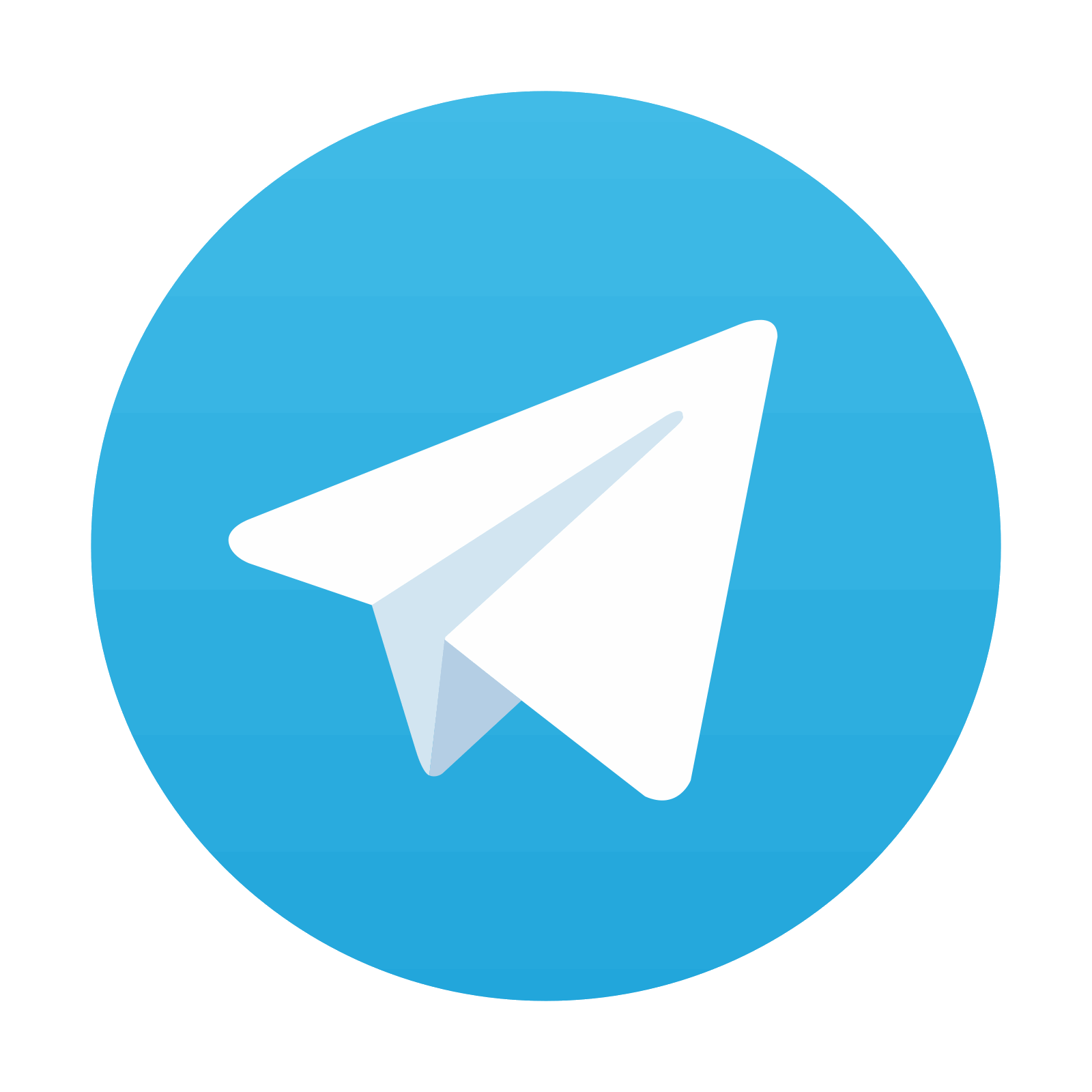
Stay updated, free articles. Join our Telegram channel
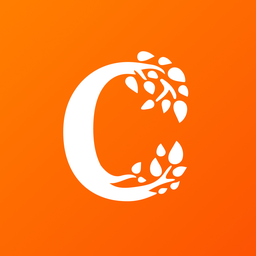
Full access? Get Clinical Tree
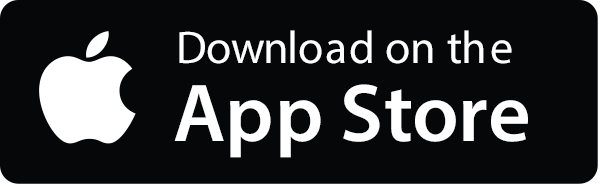
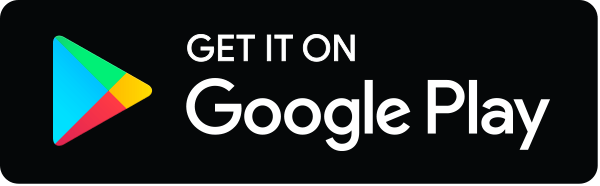