Exercise in Patients with Diabetes Mellitus
Jeanne H. Steppel
Edward S. Horton
Exercise has long been recognized as an important factor in the treatment of diabetes mellitus. Before the discovery of insulin, patients with diabetes, particular those with type 1 diabetes, were very limited in their ability to exercise, because it was almost impossible for them to avoid ketosis and dehydration. After insulin therapy was established as a mainstay treatment, exercise was no longer an elusive activity. With their ability to exercise, it became evident that hypoglycemia frequently developed both in the immediate postexercise period and during the 24 hours after exercise. It also was recognized that ketosis could be induced by exercise in patients with poor glucose control and that even patients with excellent control would sometimes develop hyperglycemia after vigorous exercise. As our understanding of exercise in the patient with type 1 diabetes has increased, the goal has been to manage glucose homeostasis and fuel metabolism so that patients can participate fully in all forms of exercise.
Exercise also plays a critical role in patients with type 2 diabetes. It can help improve insulin sensitivity and assist with reduction and maintenance of body weight in obese patients. Exercise, together with diet and pharmacologic therapies, is important as part of the overall approach to improving glycemic control and reducing cardiovascular risk factors. Indeed, exercise often is “prescribed” as a therapy for type 2 diabetes. The many benefits of exercise in these patients include improved long-term glycemic control as a result of the decrease in insulin resistance and of the cumulative blood glucose-lowering effects of individual bouts of exercise. In addition, regular exercise has been shown to improve lipid abnormalities and lower blood pressure (1,2,3). Finally, exercise also may be an important component of weight-loss regimens for these patients. When used in combination with dietary changes (especially calorie restriction), exercise promotes loss of adipose tissue with preservation of lean body mass (4,5). In addition, exercise may promote a beneficial redistribution of body fat. Abdominal adiposity appears to have a greater impact on insulin resistance than does fat deposition at other sites, and exercise has recently been shown to decrease abdominal fat in postmenopausal women (6). Unfortunately, there are some significant risks of exercise in the patient with type 2 diabetes, including symptomatic hypoglycemia, which can occur up to 24 hours after exercise; exacerbation of known or previously unknown cardiac disease; worsening of symptoms secondary to degenerative joint disease; and possible damage to joints in the setting of neuropathy. It is particularly important to screen patients with type 2 diabetes for existing cardiovascular disease before prescribing an exercise regimen.
There are several universal risks of exercise in patients with type 1 or type 2 diabetes. Most important, vigorous exercise can cause retinal hemorrhage or vitreous bleeding in patients with proliferative retinopathy. Maneuvers such as the Valsalva maneuver that increase intraabdominal pressure should be avoided, as should jarring head motions that might induce retinal detachment. In addition, patients with sensory neuropathy should refrain from high-impact exercise to reduce the risk of soft tissue and joint injury. The presence of autonomic neuropathy often makes performance of high-intensity exercise difficult because of decreased aerobic capacity and postural hypotension. Proteinuria tends to increase with exercise in patients with nephropathy. However, this is thought to merely be a result of a transient change in renal blood flow, as opposed to worsening of renal disease. Angiotensin-converting enzyme inhibitors have been shown to decrease this effect (7,8,9).
PHYSIOLOGY OF EXERCISE IN HEALTHY INDIVIDUALS
To understand metabolic regulation in patients with diabetes, it is helpful first to discuss the physiology of exercise in healthy individuals without diabetes. Several hormonal, cardiovascular, and neurologic responses that occur during exercise enable the body to respond to the increased energy demand.
In the resting fasted state, before exercise, blood glucose levels are maintained by a balance of the production of glucose by the liver and the uptake of glucose by body tissues (50% by the brain; 15% to 20% by skeletal muscle; and the remainder by kidney, splanchnic bed, blood cells, and other tissues). Glucose production by the liver early in fasting occurs predominantly via glycogenolysis, with only about 25% contributed by gluconeogenesis (Fig. 38.1). In patients with type 1 diabetes, up to 45% of glucose production during the nonexercising state derives from gluconeogenesis, even early in fasting.
The sources of energy used by skeletal muscle vary markedly between times of rest and exercise. At rest, only 10% of the energy produced in skeletal muscle is from glucose oxidation, whereas 85% to 90% is from fatty acids and 1% to 2% is from amino acids (10). Carbohydrate metabolism increases significantly with the onset of exercise as the breakdown of glycogen in muscle increases. This is associated with the rapid generation of lactate, which enters the bloodstream. Within minutes of the onset of exercise, anaerobic metabolism switches to aerobic metabolism, and uptake of glucose and oxygen into muscle increases as the blood flow to muscle increases. The circulating glucose concentration is kept essentially constant as a result of a regulated matching of the hepatic production of glucose to the rate of glucose uptake by the muscle from the circulation. In addition to the shift in glucose metabolism during exercise, breakdown of triglycerides in adipose tissue releases fatty acids into the circulation as an alternative metabolic fuel. Glycerol released from the triglyceride backbone is taken up by the liver and used as a precursor for gluconeogenesis along with the release of amino acids from the skeletal muscle.
Once exercise stops, the increase in glucose uptake continues for a time to rebuild glycogen stores in the muscle. The rate of repletion of glycogen stores can vary dramatically, depending on intake of food. This occurs quite slowly in the fasted state; in the fed state, glycogen generally is replenished within 12 hours. Glycogen stores in skeletal muscle are repleted more rapidly than are those in the liver.
Multiple complex neurologic and hormonal responses play important roles in fuel homeostasis during exercise. These include activation of the sympathetic nervous system and a change in the ratios of insulin and the counterregulatory hormones. The shifts in the balance of these hormones, plus increased sympathetic tone, alter the metabolism of glucose, free fatty acids, and amino acids and change the body’s ability to utilize oxygen and to maintain fluid status.
At the onset of exercise, the sympathetic nervous system is activated, with a resultant increase in heart rate and constriction of the blood vessels supplying the splanchnic bed, the kidneys, and muscles not involved directly in the exercise. This causes an increase in blood flow to the tissues most in need—the exercising muscles. In addition, epinephrine and norepinephrine play vital roles in stimulating breakdown of adipose tissue [β-adrenergic stimulation (11)] and suppressing insulin secretion (α-adrenergic stimulation). Catecholamines are also important in stimulating glycogenolysis during exercise.
Adjustments in insulin secretion are critical for the regulation of fuel metabolism during exercise. As mentioned above, the sympathetic nervous system suppresses secretion of insulin at the onset of exercise. Because insulin normally inhibits hepatic glucose production, the decrease in insulin allows the
liver to increase glucose output. Insulin also suppresses lipolysis, and thus the decrease in insulin levels promotes the breakdown of adipose tissue triglycerides. As will be discussed later, the molecular mechanisms of glucose uptake during exercise are independent of insulin, so the decrease in serum insulin concentration does not affect the ability of the muscle to take up glucose from the circulation (12).
liver to increase glucose output. Insulin also suppresses lipolysis, and thus the decrease in insulin levels promotes the breakdown of adipose tissue triglycerides. As will be discussed later, the molecular mechanisms of glucose uptake during exercise are independent of insulin, so the decrease in serum insulin concentration does not affect the ability of the muscle to take up glucose from the circulation (12).
Glucagon is important in the regulation of glucose levels during vigorous or prolonged exercise but plays a smaller role in mild-to-moderate exercise. As the plasma glucose concentration begins to fall, glucagon acts as a counterregulatory hormone, contributing to the activation of glycogenolysis and to the increase in gluconeogenesis through accelerated uptake of amino acids by the liver (13). The role played by glucagon is generally larger in people who have not undergone physical training than in those who are trained athletes. Cortisol and growth hormone also act as counterregulatory hormones that help to block the effects of insulin during exercise. They may be especially important in antagonizing the effects of insulin in tissues that are not directly involved in exercise, thus increasing the amount of glucose available for the actively exercising muscles.
Several factors can alter fuel utilization and the extent of influence of the hormonal and neurologic regulators. These include physical training, intensity of exercise, duration of exercise, and the diet that precedes exercise. Physical training lowers the percentage of the maximum aerobic capacity (VO2 max) that is reached when doing an equivalent amount of work. Trained individuals depend more heavily on utilization of free fatty acids than of glucose for fuel. This appears to be important in developing endurance, because muscle glycogen stores in trained individuals do not become depleted as quickly as those in untrained individuals.
The intensity of exercise, defined as a percentage of VO2 max, also influences fuel metabolism. As the intensity rises, the role of glucose in providing fuel to the exercising muscles keeps increasing (Fig. 38.2), with the importance of lipolysis decreasing. The use of amino acids remains roughly the same. Once the VO2 max is greater than 75%, carbohydrate becomes the main fuel consumed by muscle and the rate of glycogenolysis is increased.
The duration of exercise affects fuel metabolism as a consequence of a time-dependent shift from the utilization of carbohydrates to the utilization of free fatty acids. Glycogen stores become depleted after several hours of moderate continuous exercise, and lipolysis becomes the main source of fuel for exercising muscle. After depletion of glycogen stores, hepatic glucose production via gluconeogenesis is essential for maintenance of blood glucose concentrations. Sometimes in prolonged exercise such as marathon running, glycogen stores are depleted, the liver is unable to keep up with glucose requirements through gluconeogenesis, and hypoglycemia develops.
The composition of the diet preceding exercise can affect fuel metabolism during activity. A diet high in carbohydrates is associated with a greater rate of glucose oxidation and increased muscle glycogen stores. This may contribute to generally greater endurance in individuals who have had a carbohydrate-rich diet than in those with a carbohydrate-restricted diet. Some athletes therefore use a technique called “carbohydrate loading” before exercise to improve endurance.
Skeletal muscle is able to take up glucose from the circulation predominantly via the GLUT4 transporter protein (14).
During exercise, GLUT4 is translocated from an intracellular location to the plasma membrane, similar to what occurs with insulin stimulation. There is now abundant evidence to indicate that the insulin-mediated and contraction-mediated mechanisms are distinct (15). Insulin-mediated signaling involves binding of insulin to the insulin receptor and causing its autophosphorylation and a cascade of reactions, including activation of insulin receptor substrate protein-1 (IRS-1) and phosphatidylinositol 3-kinase. Exercise-induced glucose transport does not involve either IRS-1 or phosphatidylinositol 3-kinase, although there may be activation of the signaling serine kinase Akt, which is located farther down in the insulin signaling pathway.
During exercise, GLUT4 is translocated from an intracellular location to the plasma membrane, similar to what occurs with insulin stimulation. There is now abundant evidence to indicate that the insulin-mediated and contraction-mediated mechanisms are distinct (15). Insulin-mediated signaling involves binding of insulin to the insulin receptor and causing its autophosphorylation and a cascade of reactions, including activation of insulin receptor substrate protein-1 (IRS-1) and phosphatidylinositol 3-kinase. Exercise-induced glucose transport does not involve either IRS-1 or phosphatidylinositol 3-kinase, although there may be activation of the signaling serine kinase Akt, which is located farther down in the insulin signaling pathway.
Numerous hypotheses have been proposed to explain the mechanism of exercise-induced glucose transport. Calcium flux in the muscle cell has been implicated, because glucose transport decreases when calcium release is blocked pharmacologically (15). Calcium is also thought to play an important role in the activation of enzymes such as protein kinase C, which are thought to be upstream of the mobilization of GLUT4. Another theory implicates nitric oxide as a potential mediator of contraction-induced glucose transport, because generation of nitric oxide increases during exercise (16). Although the uptake of glucose by muscle after contraction is not affected by inhibition of nitric oxide synthase, basal rates of glucose transport in muscle appear to be decreased (17). It is possible that nitric oxide modulates a unique pathway that affects glucose transport independent of either the insulin or the exercise pathway.
Evidence also has implicated mitogen-activated protein (MAP) kinase in the process of exercise-induced glucose transport. The MAP kinase signaling pathway contains several enzyme cascades that are activated with exercise. This appears to include both the ERK1/2 and JNK MAP kinases (18). In addition to potential effects on glucose uptake, the MAP kinase pathways likely regulate gene-transcription events that are involved in muscle growth and repair.
There currently is strong interest in the hypothesis that 5′-adenosine monophosphate-activated protein (AMP) kinase serves as a metabolic “fuel gauge” and key regulator of glucose uptake during exercise. AMP kinase activity increases markedly with exercise. Studies with activators and inhibitors of AMP kinase indicate that this is causally linked to the translocation of GLUT4 transporters (19,20). Fatty acid oxidation and insulin sensitivity may also be affected by exercise via this pathway. Several excellent review articles have covered the signaling pathways implicated in exercise-induced glucose transport (15,21,22).
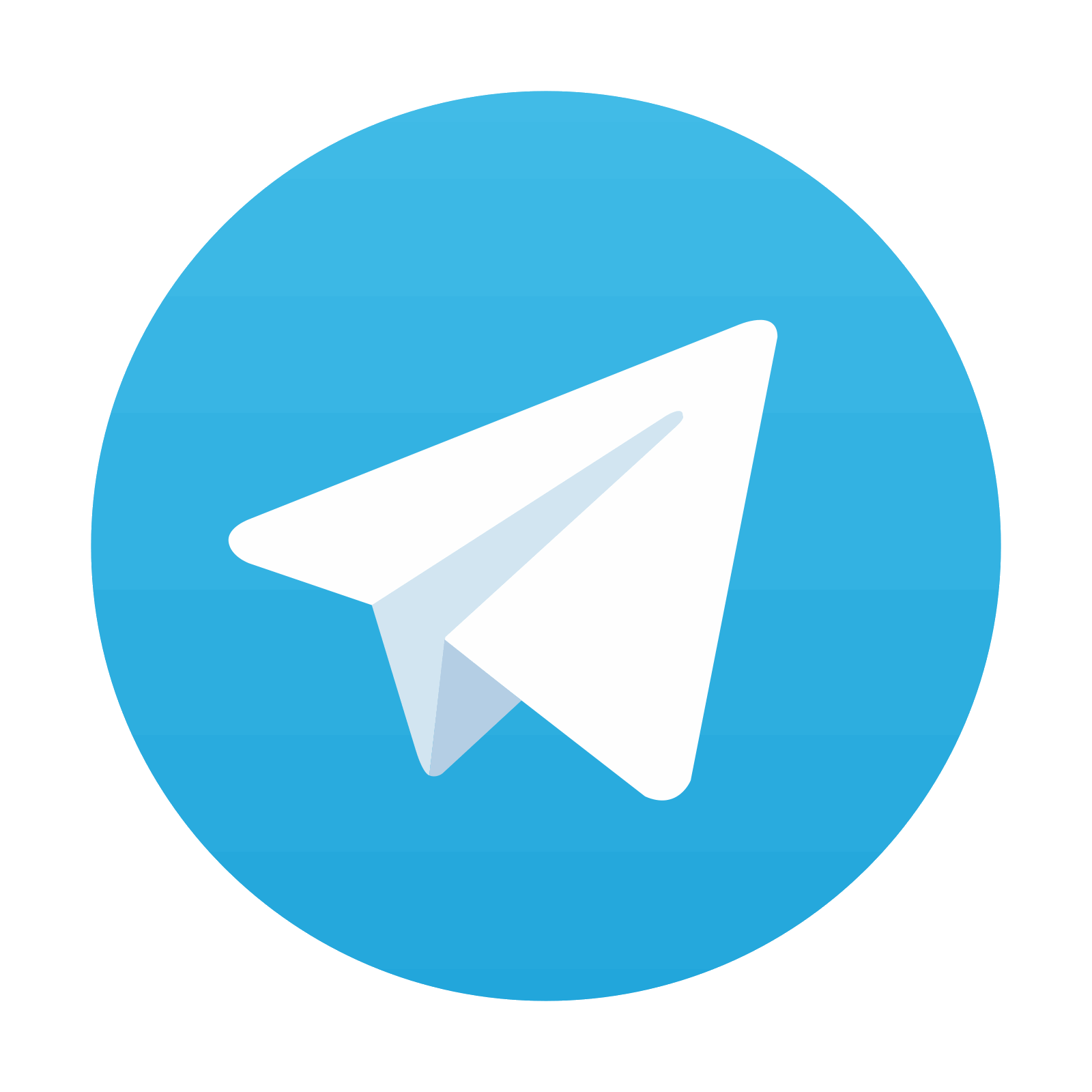
Stay updated, free articles. Join our Telegram channel
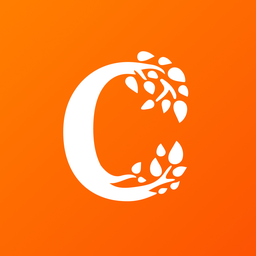
Full access? Get Clinical Tree
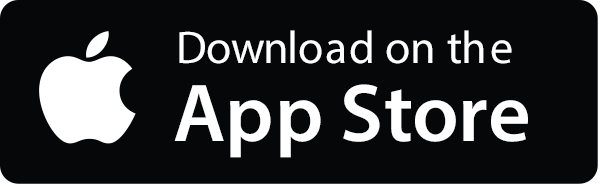
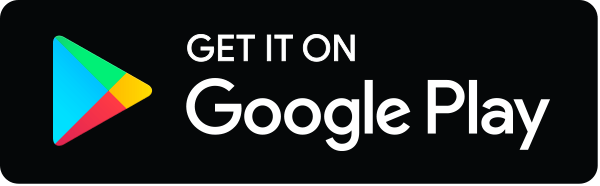