- The number of women with diabetes becoming pregnant is increasing, in line with the increase in type 2 diabetes (T2DM) among women of reproductive age. Rising obesity levels are also contributing to the increasing number of women developing glucose intolerance during pregnancy.
- Perinatal mortality and morbidity is increased in diabetic pregnancies, mainly through increased stillbirths and congenital malformations rates, which are three- to fivefold higher than in non-diabetic pregnancies.
- Metabolic changes occur in pregnancy to facilitate nutrient transfer to the fetus. Maternal insulin resistance helps divert glucose to the fetus. Women with pre-gestational diabetes have insufficient insulin to counter the rise in insulin resistance and require increasing insulin therapy during pregnancy. Women without diabetes before pregnancy become glucose intolerant in pregnancy if they have insufficient insulin reserves.
- Maternal diabetes affects all aspects of pregnancy from conception to the timing and mode of birth. The major early effects are on embryogenesis and the risk of malformations. Glycemic control is critical at this time as glucose is teratogenic to the developing embryo.
- Maternal diabetes affects fetal pancreatic islets and (β-cell development which may be a factor in the increased risk of diabetes among children of mothers with diabetes.
- Fetal insulin is an important fetal growth factor and accelerated fetal growth occurs in many diabetic pregnancies as a consequence of maternal hyperglycemia causing fetal hyperinsulinemia. This metabolic environment in later pregnancy may render the fetus susceptible to hypoxia and acidosis and ultimately stillbirth. It also increases the risk of transient neonatal hypoglycemia.
- Good maternal diabetic control lessens the risk to the fetus of malformations, accelerated fetal growth, stillbirth and neonatal hypoglycemia as well as the risk of future obesity and diabetes for the child.
- Pregnancy poses certain risks for the mother with diabetes. Any detrimental effects on retinopathy and nephropathy, however, are limited to those women with significant clinical disease beforehand and are usually self-l imiting.
- Proteinuria and microalbuminuria are risk factors for pre-eclampsia and preterm birth.
- Pregnancy in women with underlying cardiovascular disease (CVD) is associated with maternal morbidity and mortality. CVD is likely to increase as more older women with T2DM give birth.
- The increasing prevalence of maternal obesity is having an increasing impact on maternal and fetal pregnancy outcomes.
- The clinical care of women with pre-gestational diabetes and those with previous gestational diabetes mellitus (GDM) should begin before pregnancy. Women who receive pre-pregnancy care have better pregnancy outcomes and it remains a major clinical challenge to ensure that more women with diabetes are properly prepared for pregnancy.
- Preparations include the need to optimize glycemic control, to ensure folic acid supplementation and to stop all unsafe medications for pregnancy. Women should be screened for retinopathy and nephropathy before pregnancy and given relevant information concerning risks to themselves and any unborn child.
- Antenatal management should focus on achieving maternal glucose levels as near to normal as safely possible and close maternal and fetal surveillance for medical and obstetric complications. National evidence-based clinical guidelines for diabetes in pregnancy should be followed to ensure all women have access to the best available care.
- Women at risk of GDM should be screened at 28 weeks’ gestation using a 75-g oral glucose tolerance test (OGTT) and the World Health Organization criteria for impaired glucose tolerance.
- Treatment for GDM includes diet and exercise and the use of metformin, glibenclamide or insulin if glucose values cannot be maintained with diet and exercise alone.
- Both women with pre-gestational diabetes and GDM should be offered induction of labor at 38 completed weeks’ gestation to reduce the risk of a late stillbirth and the risk of birth injury in a large for gestational age infant. Insulin requirements fall to pre-pregnancy values in the immediate postpartum period and should therefore be reduced.
- The use of insulin pumps, continuous glucose monitors and the basal analog insulin is increasing in clinical practice; however, their use still needs to be fully evaluated in pregnancy.
- Following birth, early breastfeeding reduces the risk of neonatal hypogylcemia and all mothers with diabetes should be encouraged to breastfeed within 30 minutes of birth and then every 3-4 hours until a regular feeding pattern is established.
- Women with GDM should be screened for diabetes before leaving hospital, 6 weeks postpartum and then annually. In low risk
- populations, a fasting blood glucose value is sufficient and is likely to be associated with greater long-term compliance than an OGTT. All women with a history of GDM should receive lifestyle advice to lessen their long – term risk of future diabetes.
Introduction
Diabetes in pregnancy poses serious problems for both mother and fetus and has long-term health implications for the child. The number of pregnant women with pre-gestational and gestational diabetes mellitus (GDM) is increasing, mainly because of the increasing prevalence of obesity in women of childbearing age.
Despite advances in our scientific knowledge and understanding of the effects of diabetes on fetal growth and development, and improvements in fetal and maternal care, pregnancy outcomes are broadly similar to 1989, when the St. Vincent Declaration pledged to improve pregnancy outcomes to those of women without diabetes [1]. Even in highly developed health care systems, stillbirths and congenital malformations remain three- to fivefold higher than in non-diabetic pregnancies.
Improving pregnancy outcomes depends on improving glycemic control from conception to birth. Newer technologies involving glucose monitoring and insulin pumps may bring promise for the future, but the real challenge today is to ensure all women with diabetes begin their pregnancies well prepared and with optimal glycemic control.
The largest audit of diabetic pregnancies including all 3808 pregnancies of women with type 1 (T1DM) and type 2 diabetes mellitus (T2DM) who delivered or booked in 231 hospitals in England, Wales and Northern Ireland from March 1, 2002 to February 28, 2003 was published in 2005 [2]. These women accounted for 0.38% of all births at this time and provide much of the epidemiologic data in this chapter.
A confidential enquiry reviewing the demographic, social and lifestyle factors and clinical care in 521 pregnancies to women in the original audit was published subsequently [3]. This enquiry included 127 pregnancies with a fetal congenital anomaly, 95 pregnancies with fetal or neonatal death of a normally formed baby, 220 pregnancies with a good outcome and 79 pregnancies in women with T2DM and provides valuable clinical data pertaining to pregnancy outcomes.
The American Diabetic Association (ADA) [4] and the National Institute for Health and Clinical Excellence (NICE) [5] published guidelines on the clinical management of women with preexisting diabetes in pregnancy in 2008. The clinical guidelines in this chapter are based on these two consensus evidence-based guidelines.
Clinical science and epidemiology of diabetes in pregnancy
Classification of diabetes in pregnancy
The classification of diabetes is discussed in Chapter 2. A uniform classification of diabetic pregnancies is still needed for both epidemiologic and clinical purposes.
Diabetes is recognized as a heterogeneous condition [6] and while most women with pre-gestational diabetes in pregnancy will have T1DM or T2DM, a few will have monogenetic and mitochondrial forms of diabetes, while others will have secondary causes, such as cystic fibrosis. Correctly identifying the type of diabetes during pregnancy can be difficult, especially when the woman is diagnosed with diabetes during pregnancy, as some may be in the early phases of T1DM [7,8], others may have previously undiagnosed T2DM [9], while the most of the remainder have diabetes induced by pregnancy.
Pregnancy may be the first time that asymptomatic forms of monogenetic diabetes are diagnosed, as they are often present in the second and third decade [10,11]. This is true for maturity-onset diabetes of the young (MODY) caused by a mutation in the glucokinase gene (see Chapter 15). While the prevalence of this form of MODY may be less than 2% for the total antenatal population, screening selected women can increase the likelihood of finding a glucokinase gene mutation to approximately 80% in a white population. Clinical features that may suggest MODY includes a combination of:
- Fasting hyperglycemia outside pregnancy (5.5–8 mmol/L);
- A small blood glucose rise (<4.6mmol/L) during a 2-hour oral glucose tolerance test (OGTT);
- A requirement for insulin treatment during one or more earlier pregnancies but controlled on diet subsequently;
- A first-degree relative with a history of T2DM;
- GDM orfastinghyperglycemiaduringpregnancy(>5.5 mmol/L) [11].
Many of the fetal complications of a diabetic pregnancy are a direct result of maternal hyperglycemia and therefore are more dependent on glycemic control than the type of maternal diabetes. By contrast, the medical, obstetric and social risk factors differ between women with T1DM and T2DM. Understanding these differences is important for optimizing pregnancy care [3].
The CEMACH audit assessed the outcomes of 3808 pregnancies in 2767 (73%) women with T1DM and 1041 (27%) women with T2DM. Perinatal mortality, stillbirth, neonatal mortality and congenital anomaly rates were similar for both types of diabetes [2]; however, there were significant differences in pre-pregnancy planning, obesity, social deprivation, risks of recurrent and severe hypoglycemia and retinopathy, all of which may affect clinical care before and after pregnancy. Less than 1% (0.73%) of pregnancies were in women identified as either having MODY or another or unknown cause for their diabetes [2].
GDM is defined as glucose intolerance occurring or first recognized during pregnancy [12]. In the general non-diabetic antenatal population, there is no cut off between adverse pregnancy outcomes and either fasting or the 1 or 2- hour OGTT blood glucose values. The Hyperglycemia and Adverse Pregnancy Outcomes study (HAPO) performed 25 505 75-g OGTTs at 24–32 weeks’ gestation in women without diabetes and found a continuous relationship between maternal glucose concentrations and increased birth weight and increased cord-blood serum C-peptide levels; both of these measures are surrogate markers for fetal exposure to maternal glucose [13]. These associations occurred at glucose levels below those currently used to diagnose GDM.
Women who develop impaired glucose tolerance (IGT) during pregnancy, share common diabetic risk alleles [14] and have similar insulin secretory defects [15] to women with T2DM. Many of these women develop T2DM in later life [16]. For these reasons, GDM can be considered a forerunner of T2DM, with the caveat that some women diagnosed with GDM are in the preclini-cal phase of T1DM or have other rarer forms of diabetes.
Historically, the best known classification for diabetes in pregnancy was a Boston classification, named after Priscilla White who published it in 1949 [17]. The White classification was based on maternal and obstetric risk factors, graded from A (best) to F (worst) designed to predict pregnancy outcomes. Subsequent modifications to this classification were introduced in 1965 and 1971 and further updated in 1980 to incorporate ischemic heart disease and renal transplantation [18]. The White classification, like others based on purely pregnancy-related risk factors, “Prognostically Bad Signs in Pregnancy” [19], are no longer used in clinical practice.
Metabolic adaptation to pregnancy
Metabolic changes in normal pregnancy
Metabolic changes occur in pregnancy to ensure sufficient maternal fat deposition in the first half of pregnancy to sustain fetal growth in the second half of pregnancy. By the eighth week of pregnancy, fasting plasma glucose concentrations start to fall and reach a nadir by 12 weeks; by contrast, post-prandial glucose levels rise [20,21]. Fasting and glucose-stimulated insulin concentrations rise throughout pregnancy [22,23]. The fall in fasting glucose precedes changes in insulin secretion or sensitivity, and is partly caused by an increase in renal clearance of glucose early in pregnancy [24].
Maternal insulin sensitivity is reduced in late pregnancy by approximately 50%, becoming comparable with levels seen in T2DM [25–27]. For glucose tolerance to be maintained, maternal β-cells must compensate for this fall in insulin sensitivity by increasing first and second-phase insulin responses approximately threefold by the last trimester [15]. In human pregnancy, increased insulin secretion is associated with morphologic changes in the pancreas, including marked β-cell hypertrophy and hyperplasia [28]. β-Cells are dynamic and increase in both function and mass in rodent pregnancies. Whether this occurs in human pregnancy is unclear [29]. This capability is presumably decreased in women with diabetes [30].
Pregnancy-related maternal insulin resistance benefits fetal growth, because a rise in post-prandial glucose concentration aids glucose transfer to the fetus, a process termed “facilitated anabolism” [31]. Maternal to fetal glucose transfer in the fasting state is enhanced by maternal lipolysis, which occurs in late pregnancy, with free fatty acids becoming the main maternal fuel substrate and diversion of glucose to the fetus. The ability of insulin to suppress lipolysis (via inhibition of hormone-sensitive lipase in adipose tissue) is severely impaired in late pregnancy, when maternal free fatty acid release and fatty acid oxidation are increased in parallel with reduced carbohydrate oxidation [32–34]. This process of enhanced lipolysis has been termed “accelerated starvation” [35] and is attributed to the actions of human placental growth hormone and other placental hormones [36–41]. These metabolic changes facilitate the transfer of glucose and amino acids to the fetus. An increase in hepatic glucose output in late pregnancy, owing to hepatic insulin resistance, ensures that maternal glucose is available to the fetus between meals [26].
Transgenic mice that overexpress human placental growth hormone develop severe peripheral insulin resistance, similar to that found in the third trimester of pregnancy, confirming its importance in the insulin resistance of late pregnancy. This increase in insulin resistance is accompanied by an increase in expression of the p85 regulatory subunit of phosphatidylinositol kinase (PI3-kinase) and a decrease in insulin receptor substrate 1 (IRS-1) associated PI3-kinase activity in skeletal muscle [42].
The cytokine tumor necrosis factor α (TNF -α) impairs insulin signaling in vitro and is increased in the second and third trimester of human pregnancy. In late pregnancy, circulating TNF-α levels are inversely correlated with insulin sensitivity [43].
The role, if any, of other adipokines including leptin, adi-ponectin, interleukin-6 (IL-6) and resistin in insulin resistance in pregnancy still needs to be defined. Adiponectin secretion and mRNA levels in white fat decline with advancing gestation in association with decreased insulin-stimulated glucose uptake rather than altered lipid metabolism [44]. Plasma leptin doubles in pregnancy, being produced by both maternal adipose stores and the fetoplacental unit but its role in maternal metabolism is uncertain [45].
Metabolic changes in pregnancy in women with diabetes
Carbohydrate tolerance deteriorates in women with diabetes by the second trimester, in parallel with the physiologic decrease in insulin sensitivity. Women with T1DM therefore need increased insulin to maintain glycemic control from the mid-second trimester [15,46]. By contrast, in the first trimester, insulin sensitivity increases and insulin requirements at 14 weeks’ gestation are less than the start of pregnancy for well-controlled women with T1DM [46]. In 237 pregnancies in women with T1DM in Scotland, the rise in insulin requirements was associated with maternal weight at booking and weight gain between 20 and 29 weeks while inversely related to duration of diabetes [47].
The added demand on β-cell secretion may account for the increased presentation of T1DM during pregnancy [7,8]. Women in the prolonged but subclinical phase of T1DM characterized by an active insulitis may have sufficient β-cell function to prevent overt hyperglycemia before pregnancy but insufficient insulin reserve to maintain euglycemia in pregnancy.
Pregnancy-induced lipolysis makes women with T1DM more susceptible to diabetic ketoacidosis (DKA). This can develop rapidly and at relatively low levels of hyperglycemia [48]. If left untreated DKA is associated with high fetal mortality [49].
Women with pre-gestational T2DM are characteristically insulin-resistant before pregnancy with relative insulin deficiency. Further demands on their β-cells often results in a treatment escalation from diet to insulin early in pregnancy to maintain glycemic control. High doses of insulin are frequently needed, and it is not unusual for women to require in excess of 300U/day by late pregnancy, only to be well controlled with no insulin postpartum [50]. The combination of insulin resistance, decreased insulin secretion and increased lipolysis can precipitate DKA in ketosis-prone obese pregnant women with diabetes, especially among black Afro-Caribbean women, with pre-gestational T2DM or GDM [51,52].
Glucose intolerance in pregnancy
Glucose intolerance in pregnancy as defined by the WHO 1999 criteria [6] develops in a small but increasing percentage of pregnancies. These women have insufficient β-cell capacity to maintain euglycemia and are typically obese and insulin-resistant before pregnancy. They have lower insulin responses to oral glucose at 30 and 60 minutes than glucose-tolerant control subjects [53,54]. Differences in insulin sensitivity between glucose intolerant and glucose-tolerant women are less marked as pregnancy progresses [26].
Glucose intolerance in pregnancy is a continuum [13] and the higher the fasting blood glucose and 2-hour glucose value on an OGTT, the greater the underlying β-cell dysfunction and metabolic derangement. Even lesser degrees of glucose intolerance are accompanied by abnormal glycerol and free fatty acid metabolism [55] and higher circulating pro-msulin concentrations [56].
Women with a history of GDM, who become glucose-tolerant postpartum, continue to have β-cell dysfunction, reduced glucose-stimulated insulin release and impaired suppression of lipolysis [57–61]. As many of these women are obese and insulin-resistant, the persistence of decreased β-cell function postpartum increases their susceptibility to future diabetes [62–64]. Both obesity and increasing weight gain postpartum are major determinants for the development of diabetes [65].
Effect of maternal diabetes on pregnancy
Maternal diabetes influences all aspects of pregnancy, from fertility through to birth, and subsequent health of the child and adult. While hyperglycemia is the most obvious metabolic abnormality of a diabetic pregnancy, other metabolic abnormalities can also influence outcome as implied by the term “fuel-mediated tera-togenesis” [31]. In addition, diabetic complications, such as microalbuminuria, can influence the risk of obstetric complications, including hypertension and pre-eclampsia.
Placenta
A healthy pregnancy depends on a healthy placenta but maternal diabetes can cause functional and structural changes in the placenta [66–69]. Insulin receptors are highly expressed in the tro-phoblast and endothelial cells of the placenta and maternal and fetal insulin regulates nutrient transfer between the maternal and fetal circulation [70]. Placental insulin-binding capacity is increased in macrosomic diabetic pregnancies compared to non-diabetic placentas [71].
Fertility
Before the availability of insulin, most women with diabetes died within 2 years of diagnosis, and pregnancy was rare. In the 1920s, only one diabetic-related pregnancy was recorded in 35 000 deliveries at two London teaching hospitals [72]. Among those women who became pregnant approximately half of mothers and babies died [73].
Nowadays, although most young women with T1DM can be reassured about their fertility, delayed menarche in girls diagnosed before puberty is common, especially if diabetic control is poor [74,75]. More relevant to fertility is the association between T1DM and early menopause [76]. The mean self-reported age at menopause in an USA study of 143 women with T1DM diagnosed before the age of 17 years was 41.6 years, compared to 49.9 years for 186 sisters without diabetes and 48.0 years for 160 unrelated control subjects [76].
Other potential causes of infertility in women with T1DM are weight-related amenorrhea and primary ovarian failure. Eating disorders are more prevalent among young women with T1DM and are commonly associated with anovular cycles and amenor-rhea [77]. Premature ovarian failure can have an autoimmune etiology but is seldom associated with T1DM [78].
Obesity and polycystic ovarian syndrome occur more commonly in women with T2DM and both are risk factors for ano-vulatory cycles and infertility [79–81].
Miscarriage
Obtaining precise data on miscarriage rates is difficult because of selection bias, recall bias and the exact definition of the upper time limit. In the UK, miscarriage is defined as the spontaneous ending of a pregnancy before viability (currently taken as 24 weeks’ gestation). Spontaneous miscarriage rates among women with diabetes are broadly similar to the general population, which is 12–15%, although the risk is increased when diabetic control is poor [82 – 86].
Early fetal loss in non-diabetic pregnancies is often attributable to lethal chromosomal abnormalities and once a viable fetus is confirmed by ultrasound at around 8 weeks, the miscarriage rate falls and continues to fall with increasing gestational age [87,88]. Diabetic pregnancies are not at increased risk of chromosomal abnormalities but non-viable congenital malformations are more common than in non-diabetic pregnancies. These contribute to the early miscarriage rates in women with poorly controlled diabetes.
An observational UK study of 185 pregnancies in women with T1DM found a fourfold increased risk of spontaneous miscarriage when women had a glycated hemoglobin (HbA1c) level >7.5% (58 mmol/mol) compared to those with a HbA1c level <7.5% (58 mmol/mol) (7/48 vs 4/110) [89].
Maternal age and obesity are risk factors for first trimester miscarriage. A threefold increase in recurrent early miscarriage (defined as more than three successive miscarriages before 12 weeks) was found in an UK study of 1644 pregnant obese women (body mass index [BMI] > 30 kg/m2) compared with 3288 age-matched normal weight control subjects (BMI 19–24.9 kg/mm) [90]. As the number of older obese women with T2DM become pregnant, miscarriage rates are likely to rise.
Embryogenesis and malformations
Maternal hyperglycemia is a major cause of fetal malformation. Clinical and animal studies implicate a combination of metabolic, maternal and fetal factors in the etiologies of diabetic-related malformations [91]. Improved identification and classification of monogenetic and mitochondrial forms of diabetes have shown that some of these rarer forms of diabetes are associated with fetal structural abnormalities, and distinct phenotypes that are independent of maternal glycemic control have been identified (see Chapter 15).
MODY 5 diabetes, which arises from a mutation in the transcription factor hepatocyte nuclear factor-1P, exemplifies this. It is not only associated with early-onset diabetes and renal cysts, but also with a variety of other urogenital and pancreatic anomalies in the offspring [92]. There are well-documented genetic susceptibilities to glucose-mediated malformations in rodents which are likely to occur in human pregnancies [93].
Following improvements in obstetric and neonatal care, congenital malformations now represent the major cause of diabetes-related perinatal morbidity and mortality. Over the last 40 years, diabetes-related congenital malformations rates have been 4–10%. This high rate is especially poignant as good metabolic control around the time of organogenesis lessens this risk [86,94–106].
Multiple anomalies are more common in diabetic pregnancies than in non-diabetic pregnancies. This suggests the teratogenic insult occurs early in embryologic development [107,108]. While heart and CNS congenital abnormalities are the most common diabetes-related abnormalities [106]. the caudal regression syndrome (sacral agenesis) is the most pathognonomic of diabetes, being 200 times more common in diabetic pregnancies [109]. A greater than threefold excess of severe cardiac anomalies including transposition of the great arteries, truncus arteriosus and tricuspid atresia occurs in diabetic pregnancies [110].
The prevalence of major congenital anomalies in the CEMACH audit of women with pre-gestational diabetes was 41.8/1000 births [106]. This rate was twofold higher than the rate of major congenital anomalies from non-diabetic pregnancies reported as 21/1000 births in the European Surveillance of Congenital Anomalies (EUROCAT) data for 2002–2003. Anomalies of the circulatory system and neural tube were threefold higher than expected among the diabetic pregnancies.
Congenital malformations — lessons from animal studies
Rodent studies show diabetes-associated fetal malformations that are broadly similar to those of humans, although the susceptibility to particular different diabetes-related malformations depends on the maternal species and strain [93,111].
Apoptosis in the mammalian pre-implantation blastocyst is a natural process that eliminates abnormal cells. Hyperglycemia modifies the expression of key apoptotic regulatory genes and normalizing hyperglycemia in mice during the periconception period normalizes the expression of these genes [112]. In rodents, maternal hyperglycemia reduces the number of blastocysts formed and the total cell mass of those that survive. In a hyper-glycemic environment, blastocyst cell mass is reduced predominately from the inner cell layer and insulin treatment of hyperglycemic female dams, starting at the time of conception protects the blastocyst from these changes [113]. Insulin may act as a growth factor during early mammalian embryogenesis, influencing mitosis, apoptosis and differentiation through insulin receptors expressed on blastocysts [114]. Other growth factors and cytokines expressed in early blastocysts that may be involved in embryo development are the insulin-like growth factors (IGFs) and TNF-α [115].
Animal studies, predominantly in the rodent, implicate glucose as the major teratogen in diabetic pregnancies. Many of the cellular processes induce oxygen-derived free radical production and increased oxidative stress which provide a plausible unifying mechanism by which supraphysiologic concentrations of metabolic substrates, including glucose, pyruvate and hydroxybu-tyrate, could be teratogenic [91,116–118]. Hyperglycemia at the time of embryogenesis exposes the fetal mitochondria to a high influx of glucose-generated pyruvate that, by overwhelming the immature mitochondrial electron transport chain, may result in an excess of reactive oxygen species (mainly superoxide) being generated. The subsequent formation of hydrogen peroxide and hydroxyl radicals in turn leads to increased lipid peroxidation, mitochondrial swelling, DNA damage and altered expression of key genes [91]. Antioxidative agents such as N-acetylcysteine (NAC) and the vitamins E, C and folic acid protect against diabetes-induced malformations in rat embryos [27,119,120].
Myoinositol has an important role as a precursor for a number of secondary messengers and may contribute to diabetic tera-togenesis. Cultured rodent embryos in high glucose concentrations have decreased inositol uptake and become inositol deficient [121–125]. Inositol supplementation to embryos cultured in high glucose media or dietary addition to diabetic pregnant rodents protects against glucose-mediated malformation [126,127]. By contrast, the addition of an inositol uptake inhibitor to the culture medium of rodent embryos causes inositol deficiency and embryonic dysmorphogenesis, which is reversible if inositol is added to the culture [128]. Antioxidants diminish both embryonic dysmorphogenesis induced by hyperglycemia and inositol uptake inhibitors, suggesting a possible link between malformations and oxidative stress [129].
Decreased arachidonic acid and several prostaglandins, in particular PGE2, in diabetic embryogenesis have been implicated as a cause of malformations. Hyperglycemia during the period of neural tube closure reduces embryonic prostaglandin levels secondary to altered arachidonic acid metabolism and phosphati-dylinositol turnover, and the downregulation of the embryonic COX-2 gene [130]. Inhibition of rostral neural tube fusion by high glucose concentrations in rat embryos is prevented by the addition of either myoinositol or PGE2, but not if indometacin, a prostaglandin synthesis inhibitor, is also added to the culture [121]. Intraperitoneal injections of arachidonic acid to diabetic rats reduce the rates of neural tube defects (NTDs) [131]. Reduced yolk sac PGE2 concentrations have been reported from human pregnancies terminated at 8–10 weeks [132].
There is a possible link between decreased prostaglandin synthesis and oxidative stress in cultured diabetic rat embryos; the reduced PGE2 concentration seen in day-10 rat embryos and membranes exposed to high glucose concentrations can be restored when the antioxidant NAC amide is added to the culture medium. Superoxide dismutase and NAC can prevent malformations when added to the medium of rat embryos cultured in COX inhibitors, acetylsalicylic acid or high glucose concentrations [118].
NTDs in diabetic pregnancies are 2–5 times higher than in non-diabetic pregnancies [106]. Both genetic and environmental factors are involved in NTD development [133]. Although folic acid supplementation can prevent many cases of NTDs, the cellular mechanism for this protection and the relationship between maternal folate status and genetic susceptibility to NTDs are not known. Low dietary folate is an environmental factor for NTD and the 1996 dietary fortification of enriched grain products with folic acid in the USA coincided with a 20% reduction in NTDs by 1998–1999 [134]. Human studies have not shown any evidence for abnormal folate metabolism in pregnant women with diabetes [135]. In rodent studies, folic acid supplementation protects against diabetes-induced malformations [120].
Diabetic control and malformations
There is a clear association between congenital abnormalities and maternal glucose control in early pregnancy as assessed by HbA1c [2,85,89,95,101,102,136–141]. Despite the evidence that diabetic fetal malformation rates approach those of the general antenatal population when glycemic control from conception through to the end of organogenesis is tightly controlled [142144], the incidence of serious birth defects has changed little over the last few decades [103,140,145].
In a systematic review of seven cohort studies between 1985 and 2006 that examined 1977 diabetic pregnancies with 117 anomalies, the odds ratio for a congenital malformation increased by 1.2 (95% CI 1.1–1.4) for each one standard deviation (SD) unit rise in HbA1c level at the time of conception [144]. When the pre – conceptional HbA1c concentration was at the mid-normal non-diabetic range, the absolute risk of a congenital anomaly was approximately 2% (95% CI 0.0–4.4) and rose to a risk of 3% (95% CI 0.4–6.1) and 10% (95% CI 2.3–17.8) when the HbAlc levels were 2 and 8 SD above normal range, respectively.
In the CEMACH audit of pre-gestational diabetic pregnancies, 25% of women with a malformation had a first trimester HbA1c level below 53 mmol/mol (7.0%) [2]. This would suggest that at the lower levels of HbA1c this measurement of glycemic control does not assess malformation risk as well as it does at higher HbA1c values, a finding that is supported from continuous glucose monitoring studies [146].
Intensive glycemic management at the time of conception improves malformation rates [142]. In the randomized Diabetes Control and Complication Trial (DCCT), there were 191 live births from 270 pregnancies. HbA1c level at conception was significantly lower in women in the intensive treatment group than in the conventional group (57 ± 9mmol/mol [7.4% ± 1.3%] and 65 ± 5mmol/mol [8.1% ± 1.7%], respectively; P = 0.0001). There were 92 births (one set of twins) to women in the intensive glycemic management arm and 99 births (two sets of twins) to women in the conventional arm. One (0.7%) congenital malformations occurred in a woman assigned to intensive therapy compared with eight in women in the conventional arm (5.9%; P = 0.06).
Fetal pancreatic and β-cell development
Understanding the genetic and intrauterine environmental factors that influence fetal pancreatic and β-cell development and the contribution of maternal diabetes will provide insight into the trans-generational etiology of T2DM.
Immunoreactive insulin is detectable in the human fetal pancreas by 7 weeks after conception and primitive islets by 12–13 weeks, with evidence of functional fetal β-cells by the end of the first trimester [147]. Increased fetal β-cell stimulation and hyper-insulinemia in response to maternal hyperglycemia occurs in early pregnancy and may persist throughout human pregnancy. This early priming of β-cell function may explain why accelerated fetal growth patterns occur even with good metabolic control later in pregnancy [148,149].
β-Cell function and insulin sensitivity in children and young adulthood are influenced by intrauterine growth restriction [150,151]. There are critical spatiotemporal windows in embroy-onic and fetal life when maternal diet and placental function affects expression of key developmental pancreatic genes through epigenetic modifications of DNA methylation [152]. While most work has centered on models of growth restriction and subsequent β-cell development, in utero exposure to hyperglycemia also affects fetal, neonatal and adult β-cell number and function in rodents [153]; however, these experimental models may be less relevant to human pancreatic β-cell development than those in larger mammals, as the rodent fetal endocrine pancreas is more immature in utero and β-cells continue to proliferate postnatally.
The molecular changes linking growth restriction and β-cell mass in the sheep include a normal β-cell mass in fetal and early adult life, corresponding with upregulation of the genes for IGF-II and insulin gene as well as their receptors. By late adult life, however, male sheep have reduced β-cell mass and develop diabetes having lost their ability to upregulate these genes and maintain an adequate β-cell mass [154].
Pancreatic duodenal homeobox-1 (Pdx1) is a pancreatic transcription factor that regulates pancreas development and β-cell differentiation. Decreased Pdx1 expression in humans is associated with T2DM in adulthood. Reduced fetal Pdx1 expression secondary to epigenetic modification occurs in growth-restricted rodents and remains reduced into adulthood, suggesting the window for epigenetic modification of β-cell gene expression extends beyond the embryonic period [152,155].
Abnormal fetal growth
Factors influencing fetal growth include maternal–fetal nutrient transfer, maternal weight and nutritional status [156]. placental size, uterine blood flow, and fetal and parental genes [157]. In a healthy non-diabetic pregnancy, parental and fetal genes will be the major contributor to birth weight, while the fetal metabolic intrauterine environment is also a major influence in diabetic pregnancies. The infant of a mother with diabetes is often “growth promoted” [158], a finding confirmed in the CEMACH audit, in which 51% of infants were above the 90th percentile for gestational age [2]. An analysis of 643 singleton babies born after 28 weeks’ gestation to mothers with T1DM in Scotland, 1960–1999, found birth weight to be 1.41 SD above the population norm, an increase that remained unchanged over the 40 years of the study [159]. The potential consequences of accelerated growth in utero include an increased risk of emergency cesarean section, birth trauma and birth asphyxia [160,161] as well as future childhood and adult obesity.
Glucose is the major fetal and placental fuel substrate and fetal insulin and IGF-I are both fetal anabolic hormones [162], while IGF-II is a placental growth factor. Fetal insulin secretion in humans occurs in response to glucose and the amino acids arginine and leucine by 13 weeks. gestation [147,163]. Maternal hyperglycemia increases placental fetal transfer of glucose and results in fetal hyperinsulineamia [164–166]. The availability of amino acids and lactate is also increased in diabetic pregnancies [167]. The diabetic intrauterine metabolic environment promotes abdominal fat disposition and visceral growth; notably liver, spleen and heart. This type of accelerated growth pattern is visible on routine clinical ultrasound as an increased abdominal circumference from 28 weeks’ gestation (Figure 53.1) [168]. Such growth patterns are less common in hypertensive diabetic pregnancies complicated by hypertension or vascular disease which can result in decreased uterine and placental blood flow that compromises nutrient transfer [169].
Figure 53.1 Accelerated fetal growth, manifested as a progressive increase in abdominal circumference (lower panel), while head circumference remains on the 50th centile (upper panel). The red line represents the 50th centile and the shaded area represents the 3rd to 97th centile.
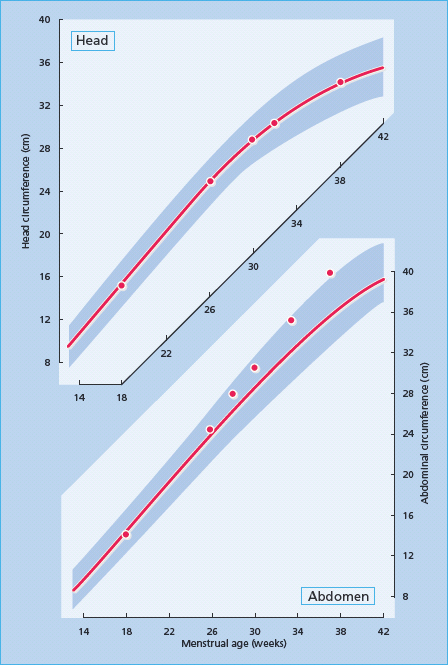
Maternal glycemic control influences fetal growth as early as the first trimester as shown in a longitudinal study on 136 pregnancies involving 120 women with diabetes in which an elevated first-trimester HbA1c level was the strongest predictor of macrosomia [170].
Accelerated growth patterns occur through maternal hyperglycemia causing fetal hyperinsulinemia, as illustrated by human and animal studies [171]. In human diabetic pregnancies there is a strong association between birth weight and fetal insulin, as assessed by amniotic and umbilical cord insulin, C-peptide and proinsulin [149,172–178].
Inducing hyperinsulinemia in the fetuses of healthy non-diabetic pregnant Rhesus monkeys produces similar accelerated fetal growth to human diabetic pregnancies [163,179]. Conversely, fetal lambs rendered hypoinsulemic in utero by streptozocin have decreased somatic and skeletal growth [180].
IGF-1 is an important fetal growth factor in humans and a homozygous IGF-I gene deletion results in severe prenatal and postnatal growth restriction and mental retardation [181]. The placental growth factor IGF-II is paternally imprinted, and functions to facilitate maternal–fetal nutrient transfer in late pregnancy, an effect that may depend on fetal insulin [182]. In healthy non-diabetic pregnancies, cord IGF-I and IGF-II are correlated with birth weight [162]. The bioavailability of the IGFs is regulated by the IGF binding proteins (IGFBPs) [183] and proteases that inhibit these binding proteins are increased in human pregnancies [184]. Cord blood IGFBP-1 is lower in T2DM and GDM compared with controls without diabetes, suggesting IGF-I bioavailability is increased in diabetic pregnancies [185].
Stillbirth
Stillbirth in the UK is currently defined as fetal loss occurring after 24 completed weeks’ gestation. In both women with and without diabetes, there are identifiable causes for stillbirth that include congenital malformations, chromosomal abnormalities, infection and intrauterine growth restriction. Approximately one-quarter of all cases are unexplained [186]. The risk of stillbirth in diabetic pregnancies is approximately fivefold higher than for non-diabetic pregnancies [101,106,140]. In the CEMACH audit, there were 63 stillbirths in 2536 births in one calendar year, representing a 4.7 (3.7–6.0) higher risk than for the general UK antenatal population that has a stillbirth rate of 5.7/1000 births. There was no difference in the stillbirth rate between T1DM (25.8/1000) and T2DM (29.2/1000). A similar stillbirth rate of 25/1000 births was seen in an earlier audit of T1DM pregnancies in the north-east of England, 1990–1994, in which there were nine stillbirths among 462 pregnancies in 355 women [102]. In a Danish cohort of 1361 consecutive singleton births to women with T1DM, 1990–1999, 1.8% resulted in stillbirth [187]. Detailed information of these pregnancies including autopsy data was available on all 25 stillbirths (22 women). No anatomical or other identifiable cause was found in 12 cases. Compared with a reference group of 236 consecutive births to women with T1DM, suboptimal glycemic control early and late in pregnancy occurred more frequently; the HbA1c level was above 58 mmol/mol (7.5%) in 64% of the stillbirth group in the first trimester and 67% in the third trimester compared with 33% and 4%, respectively, in the reference group. The CEMACH audit and other earlier studies also identified poor glycemic control throughout pregnancy as a risk factor for stillbirth [2,188]. Other identifiable risk factors in the Danish study were diabetic nephropathy, smoking and lower social status [187].
Obesity and increasing maternal age are other risk factors for stillbirth in the non-diabetic population [189,190]. Among obstetric units that serve extremely obese T2DM women (e.g. South Pacific Islanders), the stillbirth risk is higher in those with T2DM than T1DM [9,191]. As obesity and T2DM trends continue to rise among women of reproductive years, differences in stillbirth rates between women with T2DM and T1DM may become more apparent in women of Northern European ancestry.
The cause for the excess stillbirths in diabetic pregnancies that cannot be attributable to congenital malformations and other identifiable causes remains uncertain, although chronic fetal hypoxia and acidosis appear to be major contributory factors [192]. Fetal hyperinsulinemia combined with the availability of excessive fuel substrate increases oxygen demand in insulin sensitive tissues and this may exceeds placental oxygen supply [193]. Human and large animal studies suggest that the fetuses of diabetic pregnancies are more susceptible to acidosis than those of a non-diabetic pregnancy [194–196].
Amniotic erythropoietin is a marker of chronic fetal hypoxia in late pregnancy and is higher in diabetic than non-diabetic pregnancies [192]. In one study of 156 women with T1DM, amniotic erythropoietin levels correlated inversely with cord blood pH and pO2 at birth and neonatal hypoglycemia and were positively correlated with maternal HbA1c levels in late pregnancy [178]. The clinical potential of using fetal amniotic fluid erythropoietin levels to identify high-risk pregnancies needs to be assessed in clinical trials. Another potential factor that could have a minimal affect on fetal hypoxia in late diabetic pregnancies is the greater oxygen affinity of glycated hemoglobin compared with non-glycated hemoglobin which could theoretically impair oxygen delivery to the fetus.
The neonate
Neonates born to mothers with diabetes have a higher risk of being born preterm (less than 37 weeks. gestation), being large for gestational age, having a congenital malformation or birth injury and developing hypoglycemia requiring treatment. Neonatal admission to a special care baby unit is common although this often reflects hospital policy rather than clinical need [3].
Approximately half of all neonates born to women with diabetes are large for gestational age (above the 90th percentile) [2]. The increase in subcutaneous fat distribution in these infants combined with their high hematocrit gives them a typical “macrosomic” appearance with plethoric features and an obese body (Figure 53.2).
Figure 53.2 Left: A macrosomic baby born to a mother with diabetes. Right: A normal baby born to a mother without diabetes.
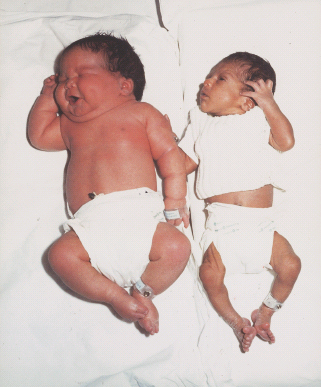
Hypoglycemia is the most common, albeit usually transient, metabolic condition in infants of women with diabetes and occurs in approximately half of all diabetic pregnancies [197]. Capillary blood glucose readings are often unreliable as many glucose sticks in routine clinical use are not quality assured and validated for neonatal use. It is therefore recommended that ward-based glucose electrode or laboratory analysis is performed to confirm hypoglycemia.
The working definition for neonatal hypoglycemia is a blood glucose value <2.6 mmol/L [198,199]. In the majority of healthy neonates, a low blood glucose concentration is merely a normal physiologic response to the metabolic adaptation to extrauterine life [200].
Macrosomic infants are at higher risk of hypoglycemia because of postpartum hyperinsulinemia secondary to in utero β-cell hyperplasia. This risk of hypoglycemia is further increased because hyperinsulinemia inhibits hepatic glucose production. Hypoglycemia may also be aggravated by polycythemia (secondary to increased glucose uptake by red blood cells), and possibly by impaired glucagon release.
Preterm infants have more variable swings in blood glucose and are therefore more at risk of hypoglycemia [201] and this may affect subsequent mental and motor development [202]. Early feeding reduces the risk of hypoglycemia [203] and it is therefore recommended that all mothers with diabetes are encouraged to breastfeed within 30 minutes of birth and then every 3–4 hours, until a regular feeding pattern is established.
The current NICE guidelines only recommend the use of intravenous glucose to treat neonatal hypoglycemia when there are clinical signs of hypoglycemia or a persistent blood sugar <2.0 mmol/L that cannot be raised by oral or tube feeding, or when the blood glucose is very low (1.1–1.4 mmol/L). If intravenous glucose is required, this should be continued until the glucose is > 2.5 mmol/L [5].
Other transient neonatal complications include polycythemia resulting from enhanced antepartum hemapoiesis [204] in response to fetal hyperinsulinemia [205] and chronic fetal tissue hypoxia [206].
Respiratory distress syndrome was considered to occur more often in diabetic pregnancies but is likely to result from preterm birth and high rate of cesarean section rather than any underlying metabolic effect [207].
Transient hypertrophic cardiomyopathy, characterized by ventricular septal hypertrophy and subaortic stenosis, occurs in up to 30% of all babies of mothers with diabetes [208,209]. Congestive cardiac failure presents in only 5% of babies and this usually has a benign course resolving within a month and without any clinical sequelae.
Both hypocalcemia and hypomagnesemia occur more frequently in the infant of a mother with diabetes, usually as a consequence of prematurity or birth asphyxia. Jaundice is more common in macrosomic babies. The cause of hyperbilirubinemia is probably multifactorial including birth trauma, polycythemia, hemolysis and immature hepatic uptake and conjugation of bilirubin.
Increased risk of diabetes in later life
Both T1DM and T2DM are the result of environmental factors operating in a genetically susceptible individual [210,211]. Diabetes in pregnancy can influence the incidence of obesity and diabetes in the offspring [212,213]. Maternal hyperglycemia during pregnancy predisposes to an earlier presentation of T2DM in the child than when diabetes develops in the mother after the pregnancy. 212]. Children and young adults of mothers with T1DM are also more insulin resistant and glucose intolerant than children of mothers without diabetes [214–216]. Similar metabolic abnormalities are not seen in the young adults of fathers with T1DM [215]. An influence of the intrauterine environment on the susceptibility to T2DM is also suggested by the observation that a family history of diabetes is more often reported on the maternal than the paternal side of the family [217–219].
Studies in the Pima population on sibling pairs born before and after the mother had developed diabetes has confirmed the importance of the intrauterine exposure to the risk of future T2DM [213]; over 70% of children with a prenatal exposure to maternal diabetes have themselves developed diabetes by 25–34 years. A prenatal exposure to maternal hyperglycemia is believed to be responsible for 40% of all T2DM in the Pima children (5–19 years) [220,221].
Growth restriction and intrauterine nutritional deprivation predispose to diabetes in later life [222]. Small-for-dates babies are at increased risk of developing T2DM or IGT in middle age.223]. suggesting that developing β-cells are programmed by intrauterine events including nutrition and hyperglycemia [224].
Risks of pregnancy for the mother with diabetes
Pregnancy may affect pre-existing microvascular and macrovas-cular disease but does not usually have any lasting detrimental effect on either retinopathy or nephropathy. In contrast, pregnancy poses a risk for women with established cardiovascular disease, and this risk should be discussed in older women and those with a long duration of disease.
Microvascular complications
Retinopathy
Diabetic retinopathy is more common in women with T1DM of childbearing age than T2DM [3]. It is not a contraindication to pregnancy but retinopathy may progress during pregnancy, especially in women with moderate or severe retinopathy before pregnancy [225,226]. Nevertheless, it rarely has any long-term detrimental effect on the natural history or progression of diabetic retinopathy [227,228]. An analysis of 59 women with T1DM before pregnancy and yearly follow-up for 5 years post pregnancy, with 10-year follow-up data available for 22 women, showed baseline retinopathy status was the only independent risk factor that predicted later progression of retinopathy [228].
Decreased retinal blood flow and retinal arterial constriction occur in the third trimester of a healthy pregnancy, but are more pronounced in diabetic pregnancies [229]. These changes, combined with pregnancy-related hypervolemia and hypercoagulation, could theoretically lower the threshold for retinal ischemia and hypoxia and hence progression of retinopathy. Pregnancy is associated with many circulating growth factors, angiopoietic factors and pro-mflammatory markers, but to date none have been clearly associated with the progression of retinopathy in pregnancy [230–233].
Retinopathy can develop de novo in pregnancy [226], although this is unlikely to progress to proliferative retinopathy [225]. Progression to proliferative retinopathy is also uncommon among women with mild or minimal retinopathy at booking. In a prospective study of 169 pregnancies among 139 women with T1DM followed between 1990 and 1998, progression to prolifera-tive retinopathy requiring laser therapy only occurred in 2.2% of pregnancies. Proliferative changes occurred in only two women, one who had occasional microaneurysms at booking and the other who had fewer than four microaneuryms per eye [225]. Progression to proliferative retinopathy requiring laser treatment is more common in women with more advanced retinopathy at booking and longer duration of diabetes [225,226,234]. Laser treatment before pregnancy protects against proliferative changes in pregnancy [226]. Hypertension influence retinopathy progres sion and optimal blood pressure control is therefore important [235].
Poor glycemic control in early pregnancy and rapid improvement of glycemic control are associated with retinopathy progression [227]. In the DCCT, 180 women with T1DM had 270 pregnancies. All women originally assigned to conventional insulin treatment were switched to intensive therapy in early pregnancy. Compared with non-pregnant control subjects in the intensively treated arm, there was a 1.6 -fold increased risk of retinopathy deterioration in pregnancy, while the deterioration in the women originally assigned to conventional treatment was 2.5-fold higher than in the non-pregnant control group. The previously conventionally treated group also had a 2.9-fold increased risk of a three-step progression or more from the baseline retinopathy level than the non-pregnant control group, with the greatest risk occurring in the second trimester (4.3-fold increase) and persisting for 12 months postpartum.
Although individual subjects had transient worsening of retin-opathy during pregnancy by the end of the DCCT, levels of retinopathy in subjects who had become pregnant were similar to those who had not, for each treatment group. The worsening of retinopathy in pregnancy in the previously conventionally treated group was attributed to the women entering pregnancy with poor glycemic control experiencing a rapid improvement in their glycemic control [227]. A similar conclusion that rapid intensification of glycemic control was a risk factor for progression of retinopathy was observed in the Diabetes in Early Pregnancy Study [234]. These studies highlight the importance of optimizing glycemic control prior to pregnancy.
Annual retinal screening with digital camera is now part of routine diabetic clinical care, and there is evidence that its introduction into clinical practice is improving the natural history of diabetic retinopathy and reducing blindness [236].
Diabetic nephropathy
Normal pregnancy causes a physiologic increase in glomerular filtration rate (GFR) and creatinine clearance. Estimated GFR formulas based on the Modification of Diet in Renal Disease (MDRD) study equation or the Cockcroft-Gault formulae are consequently unreliable during pregnancy.
Pregnancy is not associated with deteriorating kidney function in most women with diabetes. Among women with established moderate to advanced nephropathy, pregnancy may be associated with a deterioration in kidney function; however, this usually reverts to pre-pregnancy levels in the postpartum period [237].
Women with normal urinary albumin : creatinine ratios (ACR <3.5 mg/mmol) and serum creatinine (<120|imol/L) seldom experience any adverse effect on renal function during pregnancy. Women with microalbuminuria are not at risk of deteriorating renal function during pregnancy, but are at risk of pregnancy-induced hypertension, pre-eclampsia and preterm birth; early intensive hypertensive management in pregnancy may reduce these risks [238–241], as can good glycemic control [242].
Women with macroalbuminuria or proteinuria (defined as ACR >30 mg/mmol or urinary albumin concentration > 200 mg/L) are at increased risk of accelerated deterioration of renal function during pregnancy. The risk of proteinuria on maternal and fetal health increases with increasing proteinuria and declining kidney function. Pre-eclampsia is common within this group. In a prospective study of 203 women with T1DM, pre-eclampsia developed in 6% of women without microalbuminuria, in 42% with microalbuminuria and 64% of women with proteinuria [239].
Women whose protein excretion exceeds 2 g/day or whose serum creatinine concentration exceeds 120 mol/L before pregnancy require pre-pregnancy advice regarding the risk to themselves and any future unborn child. This should be given by an appropriate specialist who can provide prognostic information on diabetic nephropathy as well as advice on hypertensive management and thromboprophylaxis [5].
Overall survival rates for diabetic nephropathy continue to improve. The Danish National Register on Dialysis and Transplantation reported an increased 5-year overall survival rate of 15% between 1995 and 2005 [243]. These women need to receive realistic and current information on their kidney disease to help them make informed choices to balance a potentially shortened life expectancy and impaired quality of life with the responsibilities of motherhood.
A study of kidney function and end-stage renal replacement requirement in 93 Danish women with T1DM and diabetic neph-ropathy between 1970 and 1989 followed for 16 years found that the renal outcomes of the 26 women who became pregnant were similar to those who had not become pregnant [244]; however, nine mothers had died during the study period when their children were between 3 and 17 years old (median 9 years), and a further five required renal replacement therapy [244].
Diabetic nephropathy, especially when the serum creatinine is raised, carries a significant risk of preterm birth before 32 weeks’ gestation, very low birth weight and neonatal hypoglycemia [245]. Early intensive management of hypertension can lessen this risk [241]. The increased risk of preterm births probably accounts for the mild disturbances in growth and development in early childhood seen in offspring of these pregnancies. A 3-year follow-up study from birth of 10 children of mothers with diabetes and pre-gestational proteinuria and 30 children of mothers without proteinuria found the children of mothers with nephropathy were significantly smaller, had mild linguistic developmental delay and greater susceptibility to infections [246].
The number of women with diabetes undergoing either a renal transplant or a combined renal-pancreatic transplant is increasing. The first report of successful pregnancies following a renal transplant was in 1986 [247]. Successful pregnancies following a transplant are now common [248], with three large registries providing pregnancy outcome data. The National Transplantation Pregnancy Registry in the USA, the European Dialysis and Transplant Association Registry and the UK Transplant Pregnancy Registry all show some increased risk of miscarriages, stillbirths, ectopic pregnancies, preterm births, low birth weight babies and neonatal deaths [248].
Since 1997, the UK has had a dedicated transplant pregnancy registry and live birth rates among renal transplant recipients now approach 80% [249]. Among 176 renal transplant recipients, pregnancy was not associated with any long-term adverse effect on the transplant. When the pre-pregnancy serum creatinine was >150|imol/L, however, there was a tendency for the serum cre-atinine to be higher postpartum. The miscarriage rate, intrauterine fetal loss before 24 weeks’ gestation and stillbirth rate were 11%, 2% and 2%, respectively. Approximately half of the births occurred before 37 weeks, with a mean gestation of 35.6 weeks. Hypertension occurred in 60–80%, with pre-eclampsia diagnosed in approximately one-third, although this may be over-reported as the diagnosis of pre-clampsia is difficult in women with preexisting hypertension, renal impairment and proteinuria.
The Toronto Renal Transplant Program is one of the few studies to report follow-up data on 32 children born to renal transplant recipients. Postnatal growth was normal although developmental assessment showed one child had moderate to severe sensorineural hearing loss, another a learning disability and a third a pervasive developmental disorder [250].
It is likely in the future that more women with diabetic nephropathy will have a combined pancreas–kidney transplant over a single kidney transplantkidney transplant before a planned pregnancy. The benefit and risk of a combined pancreas–kidney transplant over a single kidney transplant has not yet been evaluated for pregnancy.
Macrovascular complications
As the general antenatal population becomes more obese and more women give birth in their late reproductive years, ischemic heart disease (IHD) in pregnancy will become more prevalent [251]. Maternal diabetes, obesity and increasing age are all identifiable risk factors for IHD in pregnancy [252,253].
Pregnancy increases the risk of an acute myocardial infarction (MI) three- to fourfold, with the risk being greatest in the peri-partum period [252]. The number of pregnant women with IHD and maternal death from IHD has increased in recent years; IHD now represents the most common cause of cardiac death associated with pregnancy in the UK [252–255]. Nevertheless, the mortality rates from acute MI in pregnancy have declined [254,255].
Women with diabetes have a twofold higher risk of dying from IHD than women without diabetes [256,257]. Population studies that capture data on 5–6% of the UK population at any one time have reported that women with T1DM, aged 35–45 years, have a 15-fold higher risk of a major CVD event [258] and women with T2DM, aged 35–54 years, have a fivefold higher risk of an MI than women without diabetes of a similar age [259].
The incidence and maternal mortality rate from acute MI in pregnancy was reported to be 1 in 35 700 deliveries and 7.3% respectively in California between 1991 and 2000 [252]; however, the mortality rate from MI among pregnant women with diabetes is likely to be higher than it is in non-pregnant women [260].
Co-morbidities
Obesity
Obesity is a common co-morbidity in women with diabetes. The antenatal population is becoming more obese, reflecting the secular trend in obesity. It has a major impact on maternal and fetal health as well as on health services and resources [261]. While one expects the majority of women with T2DM and GDM to be obese, an increasing number of women with T1DM are also overweight or obese [262]. In the CEMACH enquiry, 62% of the 137 women with T2DM and 15% of the 181 women with T1DM were obese [3].
Maternal and infant mortality [253] increases with increasing maternal BMI. The risk of congenital malformations and stillbirth are increased [263]. Obesity increases the risk of pre-eclampsia, GDM [264], the need for induction of labor, emergency cesarean section rates, postpartum hemorrhage and infection [265]. Babies of obese mothers are more likely to have birth weight above the 90th percentile [266] and be at risk of birth trauma and adult obesity and diabetes [267].
The first report linking pre-gestational weight and malformations in South Wales between 1964 and 1966 found that mothers of anencephalic infants were significantly heavier than a matched control group [268]. Subsequent epidemiologic and cohort studies have confirmed this finding. A population-based case–control study of mothers of infants born with and without selected birth defects in metropolitan areas of Atlanta, USA, between 1993 and 1997 showed for every incremental unit increase in BMI, the odds ratio for a major malformation increased by 7% [263]. The most common malformations reported occurring as either single or multiple defects, were NTDs, especially spina bifida, omphalocele and heart defects [263].
Although cardiac malformations and NTD are increased in babies of women with diabetes, obtaining euglycemia in obese women with diabetes may not fully protect them against the added risk of a congenital malformation. A cohort study of 2060 infants born to mothers with GDM showed that both diabetes severity and pre-pregnancy BMI were predictors of the 6% minor and 3.8% major malformations, with the BMI being the main contributor [269]. Data from 22 951 pregnant women enrolled in a prospective cohort study reported that major non-chromosomal congenital defects associated with diabetes were more common in obese women [270]. A study of Spanish mothers with GDM also reported that BMI >30kg/m2 was associated with a two- to threefold greater risk of cardiovascular defects compared with non-obese women [271]. Maternal obesity and GDM may increase the risk of CNS birth defects through shared metabolic mechanisms.
Autoimmune diseases
Women with T1DM are at increased risk of other autoimmune disease, especially thyroid disease and celiac disease. Thyroid function should be monitored in pregnant women with hypothy-roidism as small increases in thyroxine replacement are frequently required [272]. This is important as there is some evidence linking mild degrees of hypothyroidism in the first trimester with adverse neurodevelopment [273]. The simultaneous ingestion of iron and thyroxine may inhibit thyroxine absorption, and women should be advised to take any iron supplements at least 2 hours after or before taking thyroxine [274].
Women with a previous history of Graves disease will also need their thyroid function measured before and during pregnancy. Fetal monitoring is also recommended as maternal thyroid-stimulating antibodies can cross the placenta causing transient fetal and neonatal hyperthyroidism [275].
Clinical management
Pre-pregnancy care
Pregnancy care for women with diabetes should begin prior to conception when glycemic control can be optimized, medication reviewed and folic acid started. All health care providers who look after women with diabetes should emphasize the benefits of pre-pregnancy planning and good glycemic control on pregnancy outcomes and encourage women to plan their pregnancies and to engage in pre-pregnancy care.
Discussions about contraception and pregnancy plans should form part of the ongoing care of all pre-menopausal women with diabetes. Once a woman expresses an interest in becoming pregnant she should have access to a pre-pregnancy clinic where specific pre-conceptual advice can be given.
Evidence of effectiveness
There have been no randomized clinical trials of pre-pregnancy counseling nor are there ever likely to be. The evidence of effectiveness is therefore based predominately on observational studies of self-selected women. Furthermore, there is no standardized definition of what constitutes pre-pregnancy care [143]. Nevertheless, pregnancy outcomes are better in women with diabetes who plan their pregnancies [138]. One Scottish study analyzed adverse pregnancy outcomes by markers of pregnancy planning and pre-pregnancy care in 423 singleton T1DM pregnancies. The best pregnancy outcomes were in women who achieved an optimal HbA1c level before conceiving. These women were five times less likely to have an unfavorable outcome than women with suboptimal HbA1c levels prior to conception [276]. Pre-conception care has been shown through economic health care models to be cost effective [5,277].
Clinical studies have shown that planned pregnancies are associated with improved glycemic control in early pregnancy and fewer congenital malformations. A Dutch study of 323 women with T1DM who became pregnant showed a significantly lower incidence of major congenital malformations among the women who had planned their pregnancies (4.2% [11/271] vs 12.2% [6/52]); relative risk 0.34, (95% CI 0.13–0.88) [278].
Accessing pre-pregnancy care
Some 30–80% of women will attend a clinic for pre-pregnancy advice, but this attendance depends on the woman’ s perception of its benefits [47,279,280]. A study from Michigan, USA, found half of pregnant women with diabetes who had not received pre-pregnancy care claimed they had not been informed of its importance [279]. Women who seek out and engage in pre-pregnancy care tend to have higher educational and economic status and greater family and social support [281,282].
Unplanned pregnancies are common among women with diabetes. In the CEMACH enquiry, only 60% and 62% of women with T1DM and T2DM, respectively, had planned their pregnancy [3]. Overall, 34.5% of the women had received pre-pregnancy care: 38.2% of the women with T1DM and 24.8% of the women with T2DM.
A survey in New Zealand showed 60% of non-pregnant women with diabetes aged 18–40 years would like more information about diabetes and pregnancy [283], suggesting there is an unmet need for good pre-pregnancy advice. It remains a clinical challenge to increase the awareness and uptake of pre-pregnancy care for all women with diabetes planning pregnancy, especially among more hard to reach groups.
Information to cover d uring pre-pregnancy care
All pre-pregnancy advice should be supported by evidence of its benefit in pregnancy. The advice should be constructive and non-judgmental, and communicated in a way that is appropriate for the individual woman [284].
Women should receive information that covers the following topics:
- The benefits of optimal glycemic control and appropriate and safe glycemic targets;
- Folic acid supplementation;
- General lifestyle advice (e.g. smoking and alcohol);
- Advice on diet and exercise;
- Advice on safe prescription medications;
- General information of pregnancy risk to the woman’s health;
- General information of pregnancy risk to the fetus, neonate and their long-term health; and
- General information on diabetic pregnancy management guidelines and the need for extra surveillance.
Benefits of optimal glycemic control prior to conception
The benefits of optimal glycemic control prior to conception on pregnancy outcomes should be explained, stressing the need to continue contraception prior to achieving the agreed glycemic target. While it is important to discuss the strong association between poor pre. conception glycemic control and congenital malformations, this risk should not be exaggerated as malformations only occur in a minority of diabetic pregnancies and may discourage some women in engaging in pre-pregnancy care [280].
There is clinical evidence that pre-pregnancy care is successful in improving glycemic control in the first trimester and reduces the risk of malformation, stillbirth and neonatal death; however, it has less effect on the risk of macrosomia in later pregnancy [285]. In a meta-analysis of 14 cohort studies, the major anomaly rate was 2.1% among the 1192 offspring of women who had received preconception care compared with 6.5% in 1459 offspring of non-recipients [143]. In the nine studies that recorded both major and minor congenital anomalies, the relative risk of an anomaly with preconception care was lower (RR 0.32; 95% CI 0.17–0.59). Some of the risk may reflect important demographic differences between women who received preconception care and those who did not.
Appropriate and safe glycemic targets
Pre-conception glycemic control should be assessed by HbA1c and women should be advised to continue using contraception until their HbA1c level reaches the agreed target. An HbA1c test should be performed at 1–3 monthly intervals during this time.
The recommended pre-pregnancy HbA1c targets are lower in the UK NICE guidelines than the national pregnancy guidelines from the USA and Australia (Table 53.1).
Table 53.1 Recommended pre-pregnancy HbA1c targets in the USA, Australia and the UK.
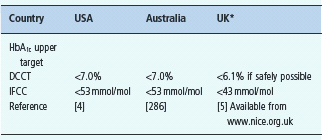
* England and Wales.
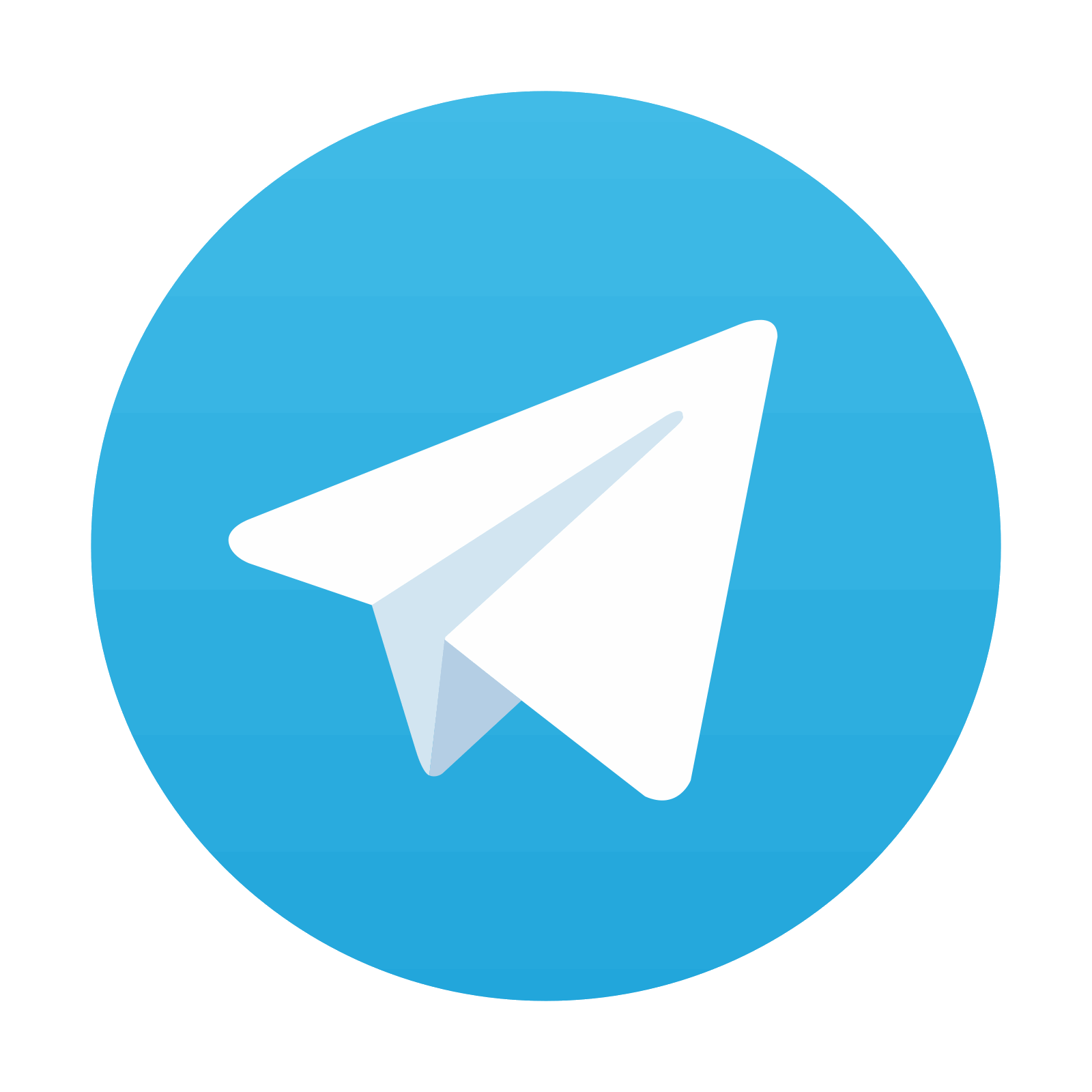
Stay updated, free articles. Join our Telegram channel
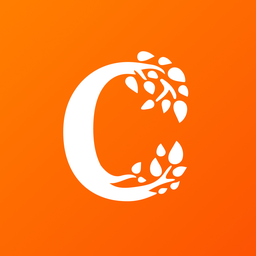
Full access? Get Clinical Tree
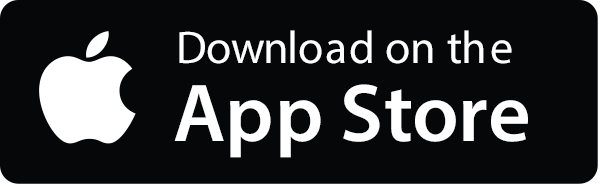
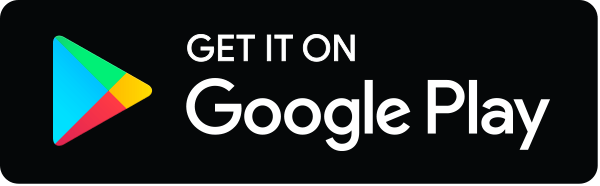