LIGANDS THAT STIMULATE SIGNAL TRANSDUCTION PATHWAYS
Receptors
The plasma membrane of eukaryotic cells serves to insulate the cell from the outside environment, but this barrier must be breached to transmit signals of extracellular origin. This fundamental problem of transmitting extracellular signals is solved in two ways. Signals cross the plasma membrane either by activating transmembrane receptors or by using ligands that are membrane permeable (Table 5.2). Cells are exquisitely sensitive to most ligands. The affinity of receptors for ligands generally is in the picomolar to nanomolar range, and very few receptors need to be occupied to transmit a signal. For example, it has been estimated that activation of ten T-cell receptors is sufficient to send a maximal signal. Cytokine-responsive cells may express only a few hundred receptors on the cell surface. Given the small number of receptors that are activated, amplification of most signals is necessary for cellular responses. A requirement for signal amplification also allows opposing or complementary pathways to affect signal strength more efficiently.2 As a result of ligand binding, receptors undergo conformational changes or oligomerization, or both, and the intrinsic activity of the receptor or of associated proteins is stimulated. Receptors may bind and respond to more than one ligand. For example, the epidermal growth factor (EGF) receptor binds to transforming growth factor-alpha (TGF-α), EGF, heparin-binding EGF (HB-EGF), beta–cellulin, epiregulin, epigen, and amphiregulin. The stimulation of most receptors leads to the activation of several downstream pathways that either function cooperatively to activate a common target or stimulate distinct targets. Generally, some of the pathways activated are counter-regulatory and serve to attenuate the signal. Receptors may also activate other receptors. A well-studied example is the activation of the EGF receptor by G protein-coupled receptors (GPCR), which occurs as a result of protease cleavage and activation of HB-EGF.
TABLE 5.2
RECEPTORS IN SIGNAL TRANSDUCTION
There are a number of transmembrane receptor families. This chapter will discuss several of them to illustrate distinct signaling mechanisms.
Receptor Tyrosine Kinases
Receptor tyrosine kinases are transmembrane proteins that have an extracellular ligand-binding domain, a transmembrane domain, and a cytoplasmic tyrosine kinase domain.3 The ligands for these receptors are proteins or peptides. Most receptor tyrosine kinases are monomeric, but members of the insulin-receptor family are heterotetramers in which the subunits are linked by disulfide bonds. Receptor tyrosine kinases have been divided into six classes, primarily on the basis of the sequence of the extracytoplasmic domain. Examples of tyrosine kinase receptors include the insulin receptor, the platelet-derived growth factor (PDGF) receptor, the EGF receptor family, and the fibroblast growth factor (FGF) receptor family.
FIGURE 5.1 Dimerization of tyrosine kinase receptors. Most tyrosine kinase receptors are activated by ligand-induced dimerization. Some ligands, such as platelet-derived growth factor (PDGF), are dimeric and induce dimerization using the two receptor-binding domains. Other ligands, such as growth hormone, contain two receptor-binding domains in the same molecule. The fibroblast growth factors (FGFs) relay on proteoglycans to aid the formation of ligand dimmers. Some ligands, such as the ephrins (EPHs), are present on nearby cells and, when the cells come into contact, bind to the receptors and promote clustering.
Activation of receptor tyrosine kinases is generally believed to require tyrosine phosphorylation of the receptor. In the case of the insulin receptor, an insulin-stimulated conformational change activates the kinase. Most of the tyrosine kinases are activated by oligomerization, which brings the kinase domains of distinct molecules into close proximity so that they cross-phosphorylate. Autotransphosphorylation of tyrosine in the activation loop of the kinase domain locks the kinase into a high-activity conformation, stimulating phosphorylation of other sites on the receptor, as well as other substrates. However, cancer-derived mutants of the EGF receptor may be activated without receptor autophosphorylation.4
Ligands stimulate receptor oligomerization in a variety of ways (Fig. 5.1). Some ligands, such as PDGF, are dimeric, so that the ligand is able to bind two receptors simultaneously.5 Other ligands, such as growth hormone, are monomeric but have two receptor-binding sites that allow them to induce receptor dimerization.6 FGFs are also monomeric but have only a single receptor-building site. FGF molecules bind to heparin sulfate proteoglycans, which concentrates FGF and facilitates dimerization of the FGF receptor.7 EGF is also monomeric, but binding of EGF to the receptor changes the receptor conformation and promotes interaction with a second ligand or receptor dimmer, leading to activation.8 Some ligand-receptor interactions result in signaling by the ligand, in addition to the receptor. Ephrins are ligands for EPH tyrosine kinase activity in the target cell, but they also stimulate signaling by ephrins in the ephrin-presenting cell.9
Studies of the EGF receptor-family illustrate some important concepts. The EGF-signaling pathways involve four receptors (EGF receptor, ERB2, ERB3, and ERB4) and many ligands.10 EGF stimulates homodimerization of the EGF receptor, but, under certain conditions, heterodimerization with other family members also occurs. Activation of EGFR proceeds via asymmetric dimerization of the receptor. Ligand causes extracellular dimerization, which then causes the kinase domains to form an intracellular head-to-tail dimer, which activates the receptor.11,12
Receptors that Activate Tyrosine Kinases
A number of receptors do not have intrinsic enzymatic activity but stimulate associated tyrosine kinases. Important examples of this type of receptor include the cytokine and interferon receptors that associate constitutively with members of the Jak family of tyrosine kinases13 and the multichain immune recognition receptors that activate SKF and Syk family tyrosine kinases.14,15 The kinase appears to be inactive in the absence of ligand, but, as happens in receptors with intrinsic tyrosine kinase activity, signaling is initiated by ligand-stimulated heterodimerization and conformational changes of the receptors.
Serine-Threonine Kinase Receptors
The TGF-β family of receptors are transmembrane proteins with intrinsic serine-threonine kinase activity.16 TGF-β ligands are dimmers that bind to and oligomerize type I and type II receptors. The type I and type II receptors homologous but distinctly regulated. The type II receptors seem to be constitutively active but do not normally phosphorylate substrates, whereas the type I receptors are normally inactive. Ligand-mediated dimerization of the type I and type II receptors causes the type II receptor to phosphorylate the type I receptor, converting it to an active kinase. Subsequent signal propagation is dependent on the kinase activity of the type I receptor and the phosphorylation of downstream substrates.
Receptor Phosphotyrosine Phosphatases
Receptor protein tyrosine phosphatases (RPTPs) have an extracellular domain, a single transmembrane-spanning domain, and cytoplasmic catalytic domains.17 The extracellular domains of some receptor tyrosine phosphatases contain fibronectin and immunoglobulin repeats, suggesting that these receptors may recognize adhesion molecules as ligands. Several RPTPs are capable of homotypic interaction, but no true ligands are yet known for RPTPs. Most receptor tyrosine phosphatases have two catalytic domains, and both are active in at least some receptors. Functional and structural evidence suggests that the phosphatase activity of some of these receptors is inhibited by dimerization. Ligand-dependent dimerization could cause constitutively active tyrosine phosphatases to lose activity, enhancing signals emanating from tyrosine kinases. RPTPs do not always function in strict opposition to tyrosine kinases, however. For example, CD45 is necessary for signaling by the B-cell receptor, which also requires tyrosine kinase activity.18 Since some Tyr-phosphorylation events, such as phosphorylation of a Tyr near the C-terminus of src-family protein-Tyr kinases, can be inhibitory to the Tyr kinase activity, activation of certain phospho-Tyr phosphatases can paradoxically cause an increase in global tyrosine phosphorylation (discussed in more detail below).
G Protein-Coupled Receptors
GPCRs are by far the most numerous receptors.19 Almost 700 GPCRs are present in the human genome.20 The number of GPCRs is so high because they encode the light, smell, and taste receptors, all of which require great diversity. These receptors have seven membrane-spanning domains: The N-terminus and three of the loops are extracellular, whereas the other three loops and the C-terminus are cytoplasmic. A wide variety of ligands bind GPCRs, including proteins and peptides, lipids, amino acids, and nucleotides. No common binding domain exists for all ligands, and interactions of ligands with GPCRs are fairly distinct.21 In the case of the thrombin receptor, thrombin cleaves the N-terminus of the receptor, freeing a new N-terminus that self-associates with the ligand pocket, leading to activation. Amines and eicosanioids bind to the transmembrane domains of their GPCRs, whereas peptide ligands bind to the transmembrane domains of their GPCRs, and peptide ligands bind to the transmembrane domains and the extracellular loops of their GPCRs. Neurotransmitters and some peptide hormones require the N-terminus for binding and activation.
Intramolecular bonds that involve residues in the transmembrane or juxtamembrane regions keep GPCRs in an inactive conformation.22 In the inactive state, the receptor is bound to a heterotrimeric G protein, which is also inactive. Agonist binding causes a conformational change that stimulates the guanine nucleotide exchange activity of the receptor. Exchange of guanosine triphosphate (GTP) for guanosine diphosphate (GDP) on the α–subunit of the heterotrimeric G proteins initiates signaling. Ultimately, GPCRs stimulate the same downstream pathways as other receptor types, including ion channels, cytosolic protein tyrosine and serine kinases, and enzymes that phosphorylate or hydrolyze membrane lipids.19 Certain GPCRs also activate receptor tyrosine kinases. As mentioned earlier, GPCR-dependent cleavage of HB-EGF stimulates the EGF receptor, which is necessary for the GPCR to activate the mitogen-activated protein kinase (MAP kinase) pathway.
Notch Family of Receptors
The Notch receptor has a large extracellular domain, a single transmembrane domain, and a cytoplasmic domain.23 Ligands for the Notch receptor are proteins expressed on the surface of adjacent cells, and activation results in two proteolytic cleavages of Notch. Initial cleavage by ADAM family proteases removes the extracellular domain and causes endocytosis. Subsequent proteolysis by the preselinin protease family releases the cytoplasmic region of Notch as a soluble signal. This fragment moves to the nucleus, where it complexes with the transcriptional repressor CBFI, relieving its inhibitory effects and stimulating transcription.
Guanylate Cyclases
Guanylate cyclases (GCs) convert guanosine triphosphate to cyclic guanosine monophosphate (cGMP) upon activation.24 There are both transmembrane and soluble forms of GCs. The membrane GCs are receptors for atrial natriuretic hormone, peptides that regulate intestinal secretion and are necessary for regulating cGMP levels for vision. In addition to the catalytic domain, the cytoplasmic tail includes a protein kinase homology domain that lacks kinase activity. Soluble GCs are activated by nitrous oxide. These receptors are widely expressed and regulate vascular tone and neuron function. They are heterodimers and each subunit has catalytic activity.
Tumor Necrosis Factor Receptor Family
The tumor necrosis factor family of receptors has a conserved cysteine-rich region in the extracellular domain, a transmembrane domain, and a domain called the death domain in the cytoplasmic tail.25 The receptors undergo oligomerization after ligand binding, which is necessary for signaling. These receptors are distinct in several respects. Stimulation of the receptor leads to recruitment of cytoplasmic proteins that bind to each other and the receptor through death domains, thereby activating a protease, caspase 8, that initiates apoptosis. Under some conditions, however, tumor necrosis factor receptors (TNFRs) stimulate antiapoptotic signals. This family of receptors also includes “decoys” or receptors that are missing all or part of the cytoplasmic tail and thus cannot transmit a signal. This feature provides a unique mechanism for inhibiting and further regulating signaling. A second class of TNFRs lack death domains but bind to TNFR-associated factors.
WNT Receptors
The Wnt family of growth and differentiation factors are small proteins that bind to cell surface receptors of the Frizzled family.26 These receptors resemble GPCRs but utilize a unique mechanism of signal transduction (Fig. 5.2). Binding of Wnt to the receptor suppresses a kinase cascade involving the protein Ser/Thr kinases casein kinase I (CK I) and glycogen synthase kinase 3 (GSK3) and the low-density lipoprotein-related protein (LRP). Active Wnt signaling requires inactivation of Axin and the adenomatous polyposis coli (APC) protein. This complex mediates phosphorylation and ultimately proteosome-dependent degradation of β-catenin. Suppression of β-catenin degradation in response to Wnt allows β-catenin to accumulate to higher levels in the cell and to migrate into the nucleus where it regulates genes involved in cell growth regulation, acting as a heterodimer with the T-cell factor (TCF) transcription factors.
FIGURE 5.2 Wingless (Wnt)/β catenin signaling. Wnt extracellular ligands bind Frizzled receptors and regulate the phosphorylation status of axin. Axin functions as part of the destruction complex that regulates the stability of β-catenin, a transcriptional regulator.
Nuclear Receptors
Ligands for nuclear hormone receptors diffuse into the cell and bind their receptors either in the cytoplasm or the nucleus. The ligands include steroids, eicosanoids, retinoids, and thyroid hormone. The sex steroids, androgens such as testosterone and estrogen and progesterone, are ligands for the androgen receptor, estrogen receptor, and progesterone receptor, respectively. Inhibition of androgen receptor is central to treatment of prostate cancer, while inhibition of estrogen receptor is central to treatment of estrogen receptor–positive breast cancer. The receptors are transcription factors that have both DNA- and ligand-binding domains. The unliganded receptor is bound to heat shock proteins that are dissociated after ligand binding. Release from the chaperone complex and ligand association lead to binding of the receptor to cofactors and DNA to regulate transcription.
Adhesion Receptors
Cell adherence, either to the extracellular matrix or to other cells, is mediated by receptors that function mechanically and stimulate intracellular signaling pathways, primarily through tyrosine kinases.27 Integrins mediate adherence to extracellular matrix and are composed of heterodimers of α and β subunits. They bind to an arginine/glycine/aspartate or leucine/aspartate/valine motif found in matrix molecules. Binding to ligand leads to integrin clustering and activation. Structural studies show that inactive integrins adopt a conformation that inhibits ligand binding. In this conformation the intracellular regions are also hindered from binding effector molecules.28 Binding of ligand opens the intracellular regions so that they bind to the molecules required to transmit integrin-dependent signals. Similarly, modification of the intercellular region, such as phosphorylation, affects the conformation of the extracellular region to favor ligand binding. This is an example of a receptor that signals both “outside-in” and “inside-out.” Integrin signaling is necessary for cell movement, but, in contrast to other pathways, adherence in nonmotile cells provides a continuous signal. This signal is necessary for survival of most cells. The ability to circumvent the requirement for adherence-dependent survival plays a major role in the development of human cancers by allowing tumor survival in inappropriate locations.
Propagation of Signals to the Cell Interior
Although the structures and mechanisms of the various receptors and ligands that initiate cell signaling are very different, most receptors activate a set of common downstream molecules to transmit their signals. The molecules that transmit signals include protein and lipid kinases, GTPases, phospholipases, proteases, adaptors, and adenylate cyclases (Table 5.3). These pathways lead to a broad array of responses, including changes in transcription and translation, enzymatic activities, and cell motility.
TABLE 5.3
ENZYME CLASSES STIMULATED BY ACTIVATED RECEPTORS
REGULATION OF PROTEIN KINASES
The balance between protein kinase and phosphatase activity controls protein phosphorylation. Protein kinases themselves, transcription factors, and cytoskeletal components are a few examples of proteins regulated by phosphorylation (Fig. 5.3). Protein kinases are classified by the residues they phosphorylate. Eukaryotic cells have protein tyrosine kinases, protein serine-threonine kinases, and dual-specificity kinases that phosphorylate serine, threonine, and tyrosine residues. Important issues in understanding the role and regulation of protein phosphorylation are how specificities or kinases and phosphatases are determined and how phosphorylation alters the function of substrates. Work at the structural and functional levels has provided preliminary answers to these questions.
FIGURE 5.3 Regulation of protein activity by phosphate. The exchange of guanosine triphosphate (GTP) for guanosine diphosphate (GDP) bound to G proteins induces an activating conformational change dependent on the additional γ phosphate of GTP. Guanine nucleotide exchange factors catalyze GDP/GTP exchange. GTPase-activating proteins (GAPs) accelerate the hydrolysis of GTP to GDP to remove the γ phosphate and attenuate G-protein signaling. Protein kinases add phosphate to proteins, resulting in conformational changes and changes in enzymatic activity. Protein phosphatases remove the phosphate to inhibit the signal. G proteins and protein kinase substrates undergo a similar cycle of phosphate addition and removal to regulate their activity. ATP, adenosine triphosphate; GEF, guanine nucleotide exchange factor.
Most signal transduction pathways require protein tyrosine kinases. Receptors that are not themselves tyrosine kinases use several cytoplasmic tyrosine kinases, including the Src, Syk, and Jak families. Phosphorylation of proteins on tyrosine can either stimulate or inhibit enzymatic activity. In addition, phosphorylation of proteins on Tyr can lead to new protein-protein interaction. An example of how tyrosine phosphorylation regulates enzymatic activity is found in the Src family of protein tyrosine kinases, which are regulated positively and negatively by tyrosine phosphorylation.29 Phosphorylation of a tyrosine residue in the C-phosphotyrosine and the Src homology 2 (SH2) domain blocks access of substrate to the catalytic domain. In contrast, phosphorylation of a tyrosine in the transactivation loop (T loop) of the catalytic domain stimulates the kinase activity by stabilizing the catalytic pocket in an active conformation.
The activity of many other tyrosine and serine-threonine protein kinases is regulated by phosphorylation of the activation, or T, loop. The T loop forms a lip of the catalytic pocket and may occlude the active site, preventing access of the substrate. In the case of the insulin receptor, the unphosphorylated T loop also appears to interfere with adenosine triphosphate (ATP) binding.30 Crystallographic studies indicate that the T loop is mobile and thus is probably not always in an inhibitory confirmation, providing kinases with some constitutive activity. Low basal activity is sufficient to phosphorylate a nearby kinase (e.g., autotransphosphorylation of a partner in a dimeric receptor). After phosphorylation, the T loop undergoes a conformational change that provides much more efficient substrate access to the catalytic site.
Once a protein kinase is active, only specific substrates are phosphorylated. Specificity is determined on two properties: colocalization of the kinase with the substrate (discussed later in this chapter) and the presence of particular motifs in a substrate that can be phosphorylated by the kinase. A proline following the serine or threonine residue to be phosphorylated is absolutely required for MAP kinase substrates. In other cases, particular motifs are favored as phosphorylation sites. These motifs probably fit best into the catalytic cleft of the kinase. In some cases, sequences distant from the site of phosphorylation mediate low-affinity association of a kinase with its substrate and thereby enhance phosphorylation.
Most signaling pathways activate serine kinases, but there is also a high level of constitutive phosphorylation of proteins on serine and threonine residues. The relevance of this basal phosphorylation is still unclear. Myriad cellular functions are regulated by serine phosphorylation, ranging from the activity of transcription factors and enzymes to the polymerization of actin. Serine kinases themselves are regulated in a variety of ways. Mammalian serine-threonine kinases have been subdivided into 11 subfamilies based on primary sequence homology, which has also been predictive of related function.31 Localization, phosphorylation, and ligand binding regulate serine kinases. Activation by ligand binding characterizes some classes of serine protein kinases. For example, cyclic nucleotides (e.g., cyclic adenosine monophosphate [cAMP]) activate the protein kinase A superfamily. Calcium and diacylglycerol (DAG) activate members of the protein kinase C (PKC) family. The protein kinase B or Akt family is activated by phosphatidylinositol (PtdIns) phosphate products of phosphoinositide-dependent kinase 1 (PDK1) to phosphorylate the activation, or T, loop. Association with cyclins activates the cyclin-dependent kinase family, and the calcium-calmodulin–dependent kinases are activated by calcium. Kinase cascades also are important in providing multiple levels of regulation and amplification of serine kinase activity. For example, MAP kinases are activated by phosphorylation of the T loop after activation of upstream kinases: Activation of Raf leads to phosphorylation and activation of MEK1, which phosphorylates and activates the extracellular signaling-regulated kinases (ERKs) (Fig. 5.4).
FIGURE 5.4 Activation of the extracellular signaling-regulated kinases (ERK) pathway. Many receptors activate the ERKs. Most receptor tyrosine kinases stimulate the activity of the Ras guanine nucleotide exchange factor son of sevenless (SOS), which associates with the linker proteins Shc and Grb2. The activation of Ras by SOS stimulates a protein serine kinase cascade initiated by Raf, which stimulates MEK. MEK then activates the ERKs. ERKs phosphorylate transcription factors to regulate gene expression. GDP, guanosine diphosphate; GPT, guanosine triphosphate.
Protein kinase signals are generally attenuated by phosphatases, metabolism of activating second messengers, or both. Dephosphorylation of the T-loop site markedly reduces the activity of most kinases, and dephosphorylation of motifs required for protein-protein binding prevents kinases from interacting with their substrates. Phosphatases also counteract the phosphorylation of substrate molecules, reversing the effects of the kinases.
Regulation of Protein Phosphatases
Protein phosphatases remove the phosphate residues from proteins, which can either activate or inhibit signaling pathways. Protein phosphatases are divided into the same three groups as the kinases on the basis of their substrates: tyrosine phosphatases, serine-threonine phosphatases, and dual-specificity phosphatases, which use a cysteinylphosphate intermediate, whereas the serine-threonine phosphatases are metal-requiring enzymes that dephosphorylate in a single step.32
Structural work has revealed how the activity of some nonreceptor tyrosine phosphatases is regulated.33 The SHP2 phosphatase has, in addition to the catalytic domain, two SH2 domains. These domains (discussed later in this chapter) mediate binding to other proteins by direct association with phosphorylated tyrosine residues. In the inactive state, the catalytic cleft of SHP2 is blocked by the N-terminal SH2 domain. Binding of the N-terminal SH2 domain to a phosphotyrosine residue of a target protein induces a conformational change that allows substrate access to the catalytic domain. Tyrosine phosphatases act to attenuate signals that require tyrosine phosphorylation and to activate pathways inhibited by tyrosine phosphorylation. An example of the negative regulatory function of tyrosine phosphatases is the role of SHP1 (a homologue of SHP2) in inhibiting cytokine and B-cell receptor signaling. In contrast, SHP2 is necessary for cytokine stimulation of cells. On the basis of the ability of phosphatase inhibitors (e.g., vanadate) to activate tyrosine kinase-dependent signaling in the absence of ligands, acute inactivation of specific tyrosine phosphatases may play an important role in regulating the balance of tyrosine phosphorylation and dephosphorylation that controls signaling pathways. Reactive oxygen generated in response to many signals can inhibit tyrosine phosphatases by oxidizing the catalytic cysteine.
Protein phosphatase 1 (PP1), PP2, PP2B, and PP2C are the major serine-threonine phosphatases; PP1 and PP2A are composed of catalytic and regulatory subunits. PP1 affects many pathways from glycogen metabolism to the cell cycle. PP2B binds to calmodulin and is regulated by calcium. Phosphorylation of either the regulatory or catalytic subunit affects the activity of serine phosphatases. More than 100 PP1 regulatory subunits function to target the catalytic domain to different cellular locations and mediate activation or inhibition. This illustrates how a single catalytic activity can perform multiple specific functions as a result of targeting by a regulatory subunit.
Guanosine Triphosphate- Binding Proteins
Protein-protein interaction is also an important mechanism of signal transduction. G proteins, which bind GTP, are the best-studied protein mediators that regulate other proteins by direct binding.21 GTP-binding proteins function as digital switches. GTP binding results in a conformational change that allows G proteins to bind to effector molecules and transmit a signal (Fig. 5.3). GTP hydrolysis to GDP ultimately returns the protein to its inactive conformation. GTP-binding proteins regulate the same molecules activated by receptors: protein and lipid kinases, phosphatases, and phospholipases. GTP-binding proteins are categorized into two large classes: the heterotrimeric GTP-binding proteins and the Ras-like GTP-binding proteins. Activation of GTP-binding proteins is regulated by guanine nucleotide exchange factors that catalyze the release of GDP and allow GTP to bind. Since the concentration of GTP in the cell far exceeds that of GDP, proteins (GAPs) accelerate GTP hydrolysis, inactivating GTP-binding proteins. All GTP-binding proteins have lipid modifications that promote membrane association.
Heterotrimeric GTP-binding proteins have three subunits and are activated by GPCRs. In the inactive state, the α, β, and γ subunits form a heterotrimer. In mammalian cells, 20 α subunits, 6 β subunits, and 12 γ subunits are known. The heterotrimeric forms are divided into four classes on the basis of function. Gαs stimulates adenylate cyclase, Gαi inhibits adenylate cyclase, and Gαq activates PLC β. G12 and G13 form a related group. In general they activate the small GTPase RhoA. GPCRs have GDP/GTP exchange activity, and binding of ligand stimulates GTP binding to the subunit of heterotrimeric G proteins. In response to GTP loading, the α and β/γ subunits dissociate. The α subunits and the β/γ complex each send signals. Release of the β/γ dimmer from the α subunit exposes surfaces that allow β/γ to bind to effectors. The α and β/γ subunits regulate a wide range of downstream effectors, including ion channels, protein kinases, and phospholipases. Domains termed regulators of G-protein signaling act as GAPs toward the α subunit and attenuate the signal by catalyzing hydrolysis of GTP to GDP.
Ras-like GTP-binding proteins are monomeric and usually of lower molecular weight than are the heterotrimeric GTP-binding proteins. Ras-like GTP-binding proteins are classified into five families: the Ras, Rho, Rab, Arf, and Ran families. The Ras and Rho families regulate cell growth, transcription, and the actin cytoskeleton; the Arf family regulates PLD and vesicle trafficking; the Rab family regulates vesicle trafficking; and the Ran family regulates nuclear import. Ras-like GTP-binding proteins are activated in a manner similar to that of the α subunit of heterotrimeric G proteins. Exchange of GTP for GDP results in a conformational change that promotes binding to the effector molecules. In contrast to heterotrimeric G proteins, nucleotide exchange for Ras-like GTP-binding proteins is not catalyzed directly by receptor or in response to specific cellular events. Signals are attenuated by the action of GAPs, analogous to regulators of the G protein-signaling domain-containing proteins that catalyze GTP hydrolysis.
GTP-binding proteins affect the activity of their targets by causing conformational changes and perhaps by serving to localize the target. Crystal structures of the catalytic domain of adenylate cyclase bound to G proteins illustrate the conformational change.34 Gαs binds to the C2a domain of adenylate cyclase, causing rotation of the C1a domain, which positions the catalytic residues more favorably for conversion of ATP to cAMP. Although crystal structures of small G proteins bound to portions of their targets also have been solved, the effect on the activity of target molecules as a result of binding has not yet been explained. Studies of the role in Ras in the interaction of Raf suggest that an important role of Ras is localization of Raf to the membrane, but Ras also may help to activate Raf directly.35
SMALL-MOLECULE SECOND MESSENGERS
Small molecules transmit signals by binding noncovalently to protein targets and affecting their function. These molecules are called second messengers because they are generated within the call in response to a first messenger, such as a growth factor, binding to a cell surface receptor.
cAMP was the first of the second messengers discovered. Adenylate cyclase, activated by heterotrimeric G proteins, catalyzed the synthesis of cAMP from ATP.36 The primary target of cAMP is protein kinase A, and the activation of protein kinase A by cAMP demonstrates how second messengers function. The inactive form of protein kinase A is a tetramer of two catalytic and two regulatory subunits; the regulatory subunit contains two cAMP-binding sites. Binding of cAMP to the first site causes a conformational change that exposes the second site. Binding of cAMP to the second site results in dissociation of the regulatory and catalytic subunits. The free catalytic subunits are then active.
Phospholipase C proteins (PLCs) are common downstream effectors of signaling.37 They cleave PtdIns-4,5-P2 to generate two small molecule signals: inositol-1,4,5-trisphosphate (IP3) and diaclyglycerol (DAG). All three families of PLC-β, –γ, and –δ are activated by calcium. PLC-β is also activated by the α and β/γ subunits of heterotrimeric G proteins, and PLC-γ is activated by tyrosine phosphorylation. DAG interacts with the C1 domain of PKCs to mediate their membrane localization and activation. IP3 binds to a calcium channel in the endoplasmic reticulum (ER) and stimulates the release in cytoplasmic calcium from intracellular stores.38 The initial increase in cytoplasmic calcium is followed by an influx of extracellular calcium via capacitive calcium channels at the plasma membrane. In unstimulated cells, cytosolic calcium is much lower than in the extracellular space of ER (100 nM vs. 1 mM), and, therefore, opening channels in the ER or plasma membrane allows calcium to flood into the cytoplasm, temporarily raising the cytoplasmic calcium to micromolar concentrations. Ultimately, calcium returns to basal levels as a result of the channels closing and removal of cytosolic calcium by both extracellular transport and pumping calcium into intracellular compartments. Calcium has a multitude of cellular effects, including directly regulating enzymatic activities, ion channels, and transcription. Several calcium-binding domains are known, including the C2 domain and EF hands. Calcium binds directly to enzymes and regulates their activity or to regulatory subunits, such as calmodulin.
Eicosanoids are ubiquitous signaling molecules that bind to GPCRs and transcription factors.39 Eicosanoid synthesis occurs in response to a number of stimuli and is an example of rapid cell-to-cell signaling. Unlike most second messengers, eicosanoids produced in one cell escape that cell and diffuse to nearby cells and either bind to receptors or are metabolized further. Eicosanoid synthesis is regulated by the production of arachidonic acid, which is produced from DAG via diglyceride lipases or from phospholipids by PLA. PLA2s clear the sn-2 acyl group of phospholipids to produce a free fatty acid and a lysophospholipid. The calcium-regulated form of PL2 shows a preference for substrates containing arachidonic acid. The further metabolism of arachidonic acid results in the synthesis of prostaglandins and leukotrienes.
The Phosphatidyl Inositol 3’ Kinase Pathway
The phosphatidyl inositol 3 (PI3)-kinase pathway is central to intracellular signaling.40,41 The intracellular messenger PIP3 is produced by PI3-kinase proteins, encoded by the PIK3CA and PIK3R1 genes for the catalytic and regulatory subunits, respectively. PIP3 is cleaved by the lipid phosphatase protein, PTEN. PI3 signaling leads to activation of the Akt kinase and the related PDK1 kinase, and then signals through the tuberous sclerosis 1 and 2 (TSC1/TSC2) complex to the mammalian target of rapamycin (mTOR) protein (Fig. 5.5).
FIGURE 5.5 The phosphoinositide 3 kinase (PI3-kinase) pathway. PI3-kinase is activated by many growth factor receptors and leads to the formation of the intracellular messenger PI3. The lipid phosphatase tumor suppressor PTEN dephosphorylates PI3K. Downstream effectors include the mammalian target of rapamycin (mTOR) kinase.
EFFICIENCY AND SPECIFICITY: FORMATION OF MULTIPROTEIN SIGNALING COMPLEXES
Compartmentalization
The ability of a signal transduction pathway to transmit a signal is dependent on the probability that a protein finds its target. The likelihood of any two proteins coming into contact is proportional to their concentrations. Recruiting a protein to a specific compartment in a cell markedly increases the local concentration of that protein, thereby enhancing the probability that it will interact with other proteins or small molecules that are recruited to or generated in the same compartment. Colocalization of proteins in a signaling pathway is achieved by recruitment to the same membrane surface or organelle (e.g., plasma membrane vs. ER) and by protein-protein interactions. Conversely, separating proteins or second messengers (or both) into distinct compartments runs off signaling pathways.
Transport of signaling proteins into the nucleus is important in a number of signal transduction pathways, and it illustrates the concept of colocalization in the same organelle.42 Nuclear transport proceeds through nuclear pores. Proteins of less than about 40 kD cross by simple diffusion, but transport of larger molecules requires a nuclear localization signal to which the importin proteins bind. The importins target the protein to the nuclear pore, and the complex is transported into the nucleus. The Ran G protein dissociates the importins from their cargo once they are in the nucleus. Regulated export of proteins from the nucleus is similar to import. A nuclear export signal is recognized by the protein exportin, which then transports the cargo out of the nucleus. A specific example is nuclear localization of the transcription factor nuclear factor of activated T cells (NFAT), which is required for its transcriptional activity.43 In response to T-cell activation and a rise in intracellular calcium, NFAT is dephosphorylated by the calcium-responsive phosphatase calcineurin. Dephosphorylation allows the nuclear localization signal in NFAT to bind to the importins, and NFAT, along with calcineurin, is imported into the nucleus. NFAT also contains a nuclear export signal, and phosphorylation appears to allow the nuclear export signal to bind to exportin, resulting in transport to the cytoplasm.
Protein compartmentalization also occurs on a smaller scale in the form of protein-protein complexes, which serve either to target proteins to particular parts of the cell or to promote efficient signal transmission. Well-studied examples of the use of protein-protein interaction to determine the localization of enzymes include the A kinase anchoring proteins that bind to protein kinase A, a family of proteins that bind to PCK, and the subunits of PP2A.44
Lipid rafts are regions where sphingolipids and cholesterol are concentrated in the outer leaflet of the plasma membrane and are important sites for signaling.45 The lipid composition provides structural cohesiveness. Rafts both concentrate and exclude proteins, promoting the formation of signaling complexes. Glycosylphosphatidylinositol-linked proteins on the extracellular surface of the plasma membrane concentrate in lipid rafts, as do acylated proteins on the intracellular surface. Transmembrane receptors can be recruited into rafts following their activation, along with their targets, leading to efficient signal generation.
Domains that Mediate Protein-Protein Binding
The regulated assembly of protein-protein complexes has several functions in signal transduction, including the formation of complexes allowing proteins to signal to each other, forming a “solid state” module that does not require diffusion to transmit a signal. Protein-protein interactions also localize an enzyme near its substrate: the binding of PLC γ1 to the PDGF receptor brings the enzyme to the plasma membrane where its substrate, PtdIns-4, 5-P2, is concentrated. These interactions are often mediated by conserved domains that recognize phosphorylated tyrosine or serine residues or proline-rich sequences (Table 5.4).
TABLE 5.4
PROTEIN-PROTEIN INTERACTION DOMAINS AND MOTIFS
SH2 and phosphotyrosine-binding domain bind to motifs that contain phosphorylated tyrosine residues.46 The crystal structures of several SH2 domains have been determined and reveal a pocket that binds the phosphotyrosine and a groove that determines binding specificity based on the fit of the residue’s C-terminal (or, in a few cases, N–terminal) to the phosphotyrosine. Tyrosine kinases and phosphatases regulate the formation of complexes involving these domains. Tyrosine kinases themselves serve as docking sites for other proteins, which is most evident with tyrosine kinase receptors that recruit PI3-kinase, p120 Ras GAP, PLCγ, and SHP-2 through SH2 domain–dependent interactions. Tyrosine kinases phosphorylate adaptors such as the IRS and Gab families of proteins also recruit other signaling molecules through phosphotyrosine-based interactions. In addition to mediating protein-protein interactions, binding of SH2 domains also bind to intramolecular phosphotyrosines, as in the case of Src, to inhibit catalytic activity.
Phosphotyrosine-binding domains are functionally analogous to SH2 domains in that they bind phosphotyrosine sequence or structural similarity to SH2 domains. Thus, they represent an independent evolutionary solution to phosphoty-photyrosine-binding, and SH2 domains bind to a tyrosine-containing motif in the absence of phosphorylation.
Recognition of phosphoserine motifs is also an important means of protein-protein interaction. Forkhead-associated domains, 14-3-3 proteins, and some WD40 and WW domains bind to regions of proteins containing phosphothreonine or phosphoserine.47 WD40 domains in members of the F-box and ubiquitination and subsequent proteolysis of proteins. A prominent example of this pathway is degradation of the inhibitor of kB (IkB), which regulates the activity of the transcription factor nuclear factor kB (NF-kB). The 14-3-3 proteins are a family of small proteins whose primary function is binding to phosphoserine or phosphothreonine motifs. An example of the importance of this interaction is the role of 14-3-3 in regulating the nuclear location of the phosphatase Cdc25 that regulates the cell cycle. Binding of 14-3-3 to phosphorylated Cdc25 leads to its export from the nucleus and blocks the cell cycle.
Src homology 3 (SH3), WW, and ena-vasp homology domains are structurally distinct, but all bind to proline-rich sequences. Many proteins that contain SH3 domains also have proline-rich regions that could be involved in intramolecular binding, suggesting that a conformational change in the protein could disrupt intramolecular binding and allow the SH3 domain to interact with other proteins. Similarly, the accessibility of proline-rich regions to SH3 domains may be regulated by conformational changes that expose the proline-rich region or disrupt an intramolecular interaction.
PDZ domains recognize motifs in the C-termini of proteins and bind to each other and lipids.48 These C-terminal motifs are found in cytoplasmic proteins, many of which also contain multiple PDZ domains. PDZ domain-containing proteins function to aggregate transmembrane proteins, such as the glutamate receptor. Group I PDZ domains bind to a consensus sequence, T/S-X-V/I, where V/I is the C-terminus of the protein. In some cases, phosphorylation of the S or T in this motif disrupts PDZ domain binding. For example, phosphorylation of this serine in the β2-adrenergic receptor was shown to lead to a loss of PDZ domain–mediated binding to EBP50, which regulates endocytic sorting of receptor.
Domains that Mediate Protein Binding to Membrane Lipids
Localization of proteins to membranes greatly limits the space in which they can diffuse and increases the probability that enzymes and substrates will contact each other. A variety of domains have evolved to bind phospholipids as a means of membrane localization (Table 5.5). C1 domains present in PKCs and some other signaling molecules bind to DAG, thereby recruiting PKCs to the membrane.49 Membrane recruitment of PKCs is also aided by the C2 domain, which binds to anionic phospholipids in the presence of calcium. This pathway is controlled by DAG production by PLC-dependent hydrolysis of PtdIns-4,5-P2.
TABLE 5.5
DOMAINS THAT BIND PHOSPHOLIPIDS
Several different domains bind to phosphoinositides, localizing the proteins that contain them to membranes.50 These domains include pleckstrin homology (PH), Phox, FYVE, FERM, and ENTH domains. Particular PH domains bind specific phosphoinositides, including PtdIns-3-P, PtdIns-4,5-P2, PtdIns-3,4-P2, and PtdIns-3,4,5-P3. Phox and FYVE domains typically bind to PtdIns-3-P. FERM and ENTH domains bind to PtdIns-4,5-P2. The accessibility of the domain and the availability of PtdIns phosphates regulate these interactions. Phosphoinositide kinases synthesize phosphoinositides. PtdIns4-kinases synthesize PtdIns-4-P from PtdIns. Type I PtdIns phosphate kinases phosphorylate PtdIns-4-P at the 5 position to make PtdIns-4,5-P2. Phosphoinositide levels are also regulated by phosphatases. PTEN and related phosphatases remove the phosphate from the 3 position of the PtdIns-3,4,5-P3. PtdIns-3,4-P2, and PtdIns-3-P.51 PTEN thus counteracts PI3-kinase signals, and cells lacking PTEN expression have increased signaling through PI3-kinase–dependent pathways. A family of phosphatases that removes the phosphate from the 5 position of PtdIns-3,4,5-P3, the SH2 inositol phosphatases (SHIP1 and SHIP2), also regulates phosphoinositide signaling pathways.52
Acute production of specific phosphoinositides in a membrane compartment results in the recruitment of proteins containing PH domains that recognize that phosphoinositide. Colocalization of a subset of proteins allows them to interact more efficiently. An example of the role of PH domains in such a pathway is the activation of Akt by PDK1.53 PDK1 and Akt are protein serine-threonine kinases that contain PH domains that bind PtdIns-3,4-P2 or PtdIns-3,4,5-P3. Activation of PI3-kinase leads to local synthesis of PtdIns-3,4-P2 and PtdIns-3,4,5-P3, which causes recruitment of Akt and PDK1 to the same membrane location. This localization facilitates phosphorylation and activation of Akt by PDK1.
Regulation of Protein Levels: Transcription, Translation, and Proteolysis
In addition to influencing the activity of proteins in the cell, signal transduction pathways also regulate the type and levels of proteins expressed in cells. This sort of regulation is necessary for development, differentiation, and the specific function of distinct cell types. Whether a protein is expressed at all in a cell is regulated at the transcriptional level, whereas transcription, translation, and proteolysis have a role in determining the concentration of a protein in a cell.
Ultimately, most signal transduction pathways regulate gene transcription and, thus, the level and type of proteins expressed in the cell. Analysis of the effects of stimuli on gene expression profiles using microarray analysis has shown that a single stimulus affects the transcription of hundreds of genes. The ability to transcribe a gene is regulated at multiple levels, including the structure of chromatin in the region of the gene, modifications of the promoter regions, and the activity of transcription factors and coactivators. Signal transduction pathways regulate histone acetylases and deacetylases that determine the accessibility of chromatin to the transcriptional apparatus. Recent work has shown that a number of signals lead to histone hyperacetylation that disrupts the nucleosome to allow transcription. These pathways cooperate with the activation of transcription factors.
Signal transduction pathways regulate transcription factors in numerous ways. The binding of ligands to the nuclear receptor family of transcription factors causes dissociation of the receptor from a complex with heat shock proteins and allows the receptor to bind to DNA. Tyrosine phosphorylation of the STAT family of transcription factors by Jak kinases in response to stimulation of cytokine receptors allows them to dimerize through their SH2 domains, enter the nucleus, and bind to DNA.54 TGF-β receptors activate transcription by phosphorylating SMAD proteins on serine residues. Phosphorylation of SMAD proteins promotes heterodimerization with SMAD4 and exposes the DNA-binding domain. Activated SMADs translocate to the nucleus complex with a protein called Fast1 and bind to DNA to regulate transcription.
Activation of transcription factors also occurs much farther downstream from the receptor. Stimulation of the transcriptional activity of Elk-1 by EGF requires a Ras exchange factor, which leads to activation of Ras. Active Ras stimulates Raf. Raf in turn phosphorylates and activates MEK1, which phosphorylates and activates ERK. Active ERK translocates to the nucleus and phosphorylates Elk-1.
Translation is controlled at several levels.55 The sequences of some RNAs result in stable tertiary structures that bind proteins to regulate location or translation. The ability of these types of RNAs to be translated is regulated by protein kinase cascades. A common mechanism is phosphorylation of initiation factor eIF-4E. p70S6 kinase regulates the translation of specific RNAs containing a 5’ terminal oligopyrimidine tract by phosphorylation of the ribosomal S6 protein. This increases the ability of the ribosome to process such messages.
The levels of some proteins are regulated by proteolysis, which occurs either via the proteosome or the lysosome. Ubiquitination targets proteins to the proteosome but can also regulate other aspects of protein function.56 An example of the role of ubiquitination is the regulation of IkB levels (introduced previously). Phosphorylation of IkB is stimulated by a number of receptor-mediated signaling pathways and leads to its dissociation from NF-kB and allows NF-kB to enter the nucleus and bind DNA. After phosphorylation, the β–transducin repeat-containing protein binds to IkB, recruiting ubiquitin ligase that catalyzes the ubiquitination of IkB and leads to its recognition and degradation by the proteosome.
The second major pathway of protein degradation is the lysosomal pathway. An early response to the stimulation of receptors is their internalization into endosomes. Some receptors continue to signal following endocytosis.57 In the case of receptor tyrosine kinases, ligand-dependent kinase activity is necessary for endocytosis, mediated by clathrin-coated pits. After endocytosis, receptors recycle to the plasma membrane of the endosomes and fuse with lysosomes, leading to degradation of the receptor.
SIGNALING NETWORKS
Although signaling pathways are usually depicted as linear cascades, nearly all signaling pathways are highly interconnected and form networks that allow dynamic regulation of the timing, strength, and duration of signaling. In addition, both feed forward and feed backward loops provide the means to self-regulate signaling or to integrate signaling from multiple signals simultaneously.
As a consequence, the same signal may induce different outcomes depending on the particular cell or cell state. For example, TGFβ can stimulate cell proliferation or arrest in different cells. With the development of small molecule and antibody inhibitors that show exquisite specificity for their targets, inhibition of one part of a signaling cascade may lead to cell death in some contexts and paradoxical proliferation in others. For example, a small molecule inhibitor of the serine-threonine kinase BRAF in some melanomas that harbor an activated mutant BRAF leads to cell death, while this same inhibitor can lead to increased proliferation in tumor cells that contain a normal BRAF gene but instead have an activating mutation in KRAS.58–60 Thus, deciphering specific pathways as well as their interconnections is necessary to understand how cells and tissues respond to physiologic and pathologic stimuli as well as to predict the response to therapy.
Selected References
The full list of references for this chapter appears in the online version.
1. Kao J, Rosenstein BS, Peters S, Milano MT, Kron SJ. Cellular response to DNA damage. Ann N Y Acad Sci 2005;1066:243.
2. Ferrell JE Jr. Self-perpetuating states in signal transduction: positive feedback, double-negative feedback and bistability. Curr Opin Cell Biol 2002;14:140.
3. Schlessinger J. Cell signaling by receptor tyrosine kinases. Cell 2000;103:211.
4. Rothenberg SM, Engelman JA, Le S, et al. Modeling oncogene addiction using RNA interference. Proc Natl Acad Sci U S A 2008;105:12480.
5. Fretto LJ, Snape AJ, Tomlinson JE, et al. Mechanism of platelet-derived growth factor (PDGF) AA, AB, and BB binding to alpha and beta PDGF receptor. J Biol Chem 1993; 268:3625.
6. de Vos AM, Ultsch M, Kossiakoff AA. Human growth hormone and extracellular domain of its receptor: crystal structure of the complex. Science 1992;255:306.
7. Spivak-Kroizman T, Lemmon A, Dikic A, et al. Heparin-induced oligomerization of FGF molecules is responsible for FGF receptor dimerization, activation, and cell proliferation. Cell 1994;79:1015.
8. Schlessinger J. Ligand-induced, receptor-mediated dimerization and activation of EGF receptor. Cell 2002;110:669.
10. Harris RC, Chung E, Coffey RJ. EGF receptor ligands. Exp Cell Res 2003;284:2.
11. Zhang X, Pickin KA, Bose R, et al. Inhibition of the EGF receptor by binding of MIG6 to an activating kinase domain interface. Nature 2007;450:741.
12. Zhang X, Gureasko J, Shen K, Cole PA, Kuriyan J. An allosteric mechanism for activation of the kinase domain of epidermal growth factor receptor. Cell 2006;125:1137.
13. Kerr IM, Costa-Pereira AP, Lillemeier BF, Strobl B. Of JAKs, STATs, blind watchmakers, jeeps and trains. FEBS Lett 2003;546:1.
14. Mustelin T, Abraham RT, Rudd CE, Alonso A, Merlo JJ. Protein tyrosine phosphorylation in T cell signaling. Front Biosci 2002;7:d918.
15. Gauld SB, Dal Porto JM, Cambier JC. B cell antigen receptor signaling: roles in cell development and disease. Science 2002;296:1641.
16. Shi Y, Massague J. Mechanisms of TGF-beta signaling from cell membrane to the nucleus. Cell 2003;113:685.
17. Tonks NK. Protein tyrosine phosphatases: from genes, to function, to disease. Nat Rev Mol Cell Biol 2006;7: 833.
19. Pierce KL, Premont RT, Lefkowitz RJ. Seven-transmembrane receptors. Nat Rev Mol Cell Biol 2002;3:639.
21. Wettschureck N, Offermanns S. Mammalian G proteins and their cell type specific functions. Physiol Rev 2005;85:1159.
24. Murad F. Shattuck lecture. Nitric oxide and cyclic GMP in cell signaling and drug development. N Engl J Med 2006; 355:2003.
26. van Amerongen R, Nusse R. Towards an integrated view of Wnt signaling in development. Development 2009;136:3205.
27. Arnaout MA, Mahalingam B, Xiong JP. Integrin structure, allostery, and bidirectional signaling. Annu Rev Cell Dev Biol 2005;21:381.
29. Bjorge JD, Jakymiw A, Fujita DJ. Selected glimpses into the activation and function of Src kinase. Oncogene 2000;19: 5620.
30. Hubbard SR. Protein tyrosine kinases: autoregulation and small-molecule inhibition. Curr Opin Struct Biol 2002; 12:735.
31. Manning G, Whyte DB, Martinez R, Hunter T, Sudarsanam S. The protein kinase complement of the human genome. Science 2002;298:1912.
32. Barford D, Das AK, Egloff MP. The structure and mechanism of protein phosphatases: insights into catalysis and regulation. Annu Rev Biophys Biomol Struct 1998;27:133.
33. Barford D, Neel BG. Revealing mechanisms for SH2 domain mediated regulation of the protein tyrosine phosphatase SHP-2. Structure 1998;6:249.
34. Simonds WF. G protein regulation of adenylate cyclase. Trends Pharmacol Sci 1999;20:66.
35. Chong H, Vikis HG, Guan KL. Mechanisms of regulating the Raf kinase family. Cell Signal 2003;15:463.
37. Rhee SG. Regulation of phosphoinositide-specific phospholipase C. Annu Rev Biochem 2001;70:281.
39. Soberman RJ, Christmas P. The organization and consequences of eicosanoid signaling. J Clin Invest 2003;111:1107.
40. Bunney TD, Katan M. Phosphoinositide signalling in cancer: beyond PI3K and PTEN. Nat Rev Cancer 2010;10:342.
41. Grant S. Cotargeting survival signaling pathways in cancer. J Clin Invest 2008;118:3003.
42. Lei EP, Silver PA. Protein and RNA export from the nucleus. Dev Cell 2002;2:261.
43. Hogan PG, Chen L, Nardone J, Rao A. Transcriptional regulation by calcium, calcineurin, and NFAT. Genes Dev 2003; 17:2205.
44. Virshup DM. Protein phosphatase 2A: a panoply of enzymes. Curr Opin Cell Biol 2000;12:180.
46. Schlessinger J, Lemmon MA. SH2 and PTB domains in tyrosine kinase signaling. Sci STKE 2003;2003(191):RE12.
47. Yaffe MB, Elia AE. Phosphoserine/threonine-binding domains. Curr Opin Cell Biol 2001;13:131.
48. Nourry C, Grant SG, Borg JP. PDZ domain proteins: plug and play! Sci STKE 2003;2003(179):RE7.
50. Hurley JH. Membrane binding domains. Biochim Biophys Acta 2006;1761:805.
51. Maehama T, Taylor GS, Dixon JE. PTEN and myotubularin: novel phosphoinositide phosphatases. Annu Rev Biochem 2001;70:247.
52. Rohrschneider LR, Fuller JF, Wolf I, Liu Y, Lucas DM. Structure, function, and biology of SHIP proteins. Genes Dev 2000;14:505.
53. Mora A, Komander D, van Aalten DM, Alessi DR. PDK1, the master regulator of AGC kinase signal transduction. Semin Cell Dev Biol 2004;15:161.
54. Levy DE, Darnell JE Jr. Stats: transcriptional control and biological impact. Nat Rev Mol Cell Biol 2002;3:651.
55. Dever TE. Gene-specific regulation by general translation factors. Cell 2002;108:545.
56. Kerscher O, Felberbaum R, Hochstrasser M. Modification of proteins by ubiquitin and ubiquitin-like proteins. Annu Rev Cell Dev Biol 2006;22:159.
57. Di Fiore PP, De Camilli P. Endocytosis and signaling. an inseparable partnership. Cell 2001;106:1.
58. Hatzivassiliou G, Song K, Yen I, et al. RAF inhibitors prime wild-type RAF to activate the MAPK pathway and enhance growth. Nature 2010;464:431.
59. Poulikakos PI, Zhang C, Bollag G, Shokat KM, Rosen N. RAF inhibitors transactivate RAF dimers and ERK signalling in cells with wild-type BRAF. Nature 2010;464:427.
60. Heidorn SJ, Milagre C, Whittaker S, et al. Kinase-dead BRAF and oncogenic RAS cooperate to drive tumor progression through CRAF. Cell 2010;140:209.
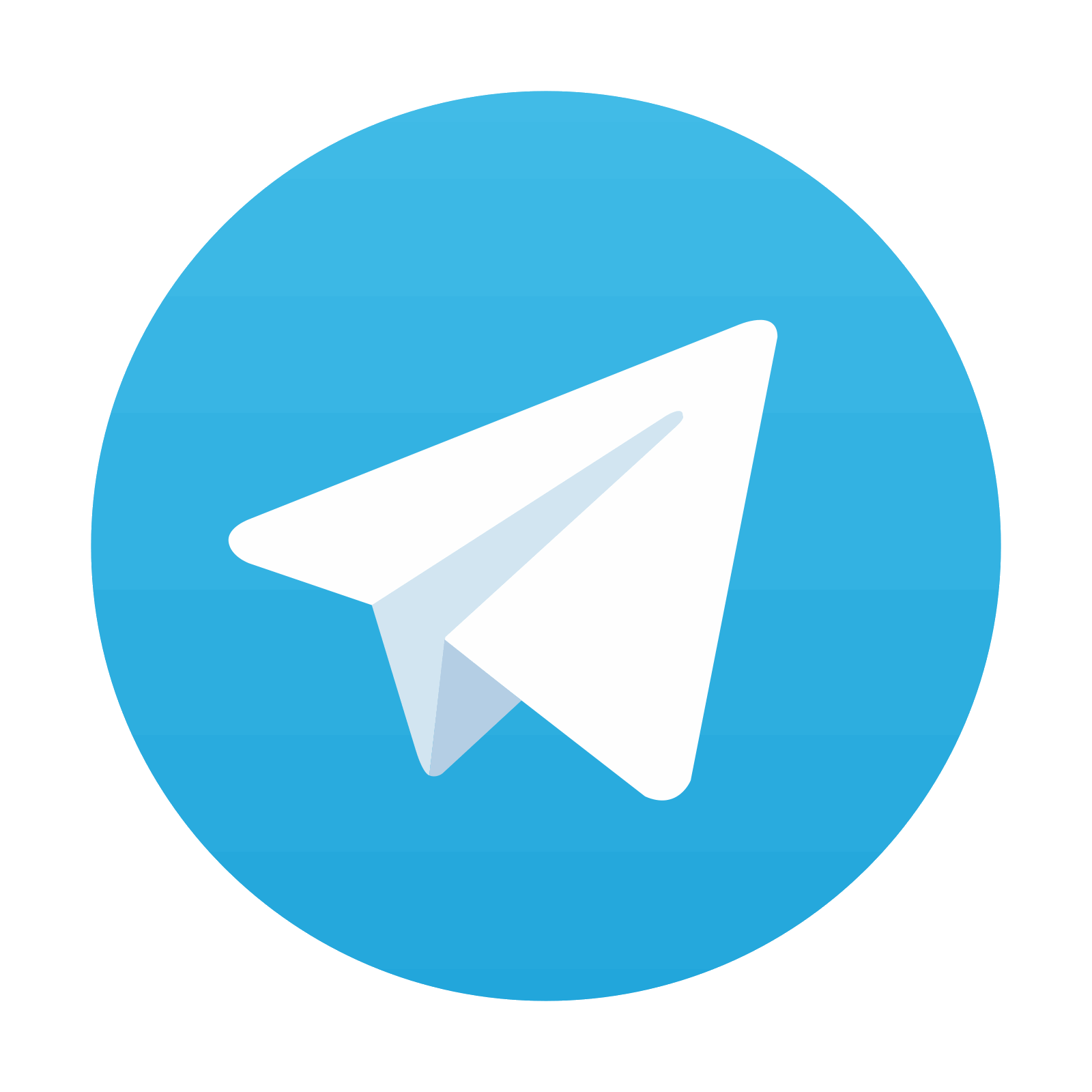
Stay updated, free articles. Join our Telegram channel
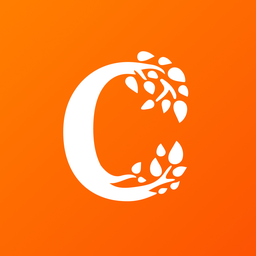
Full access? Get Clinical Tree
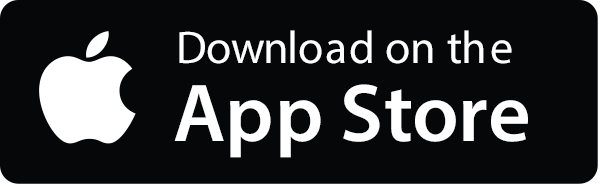
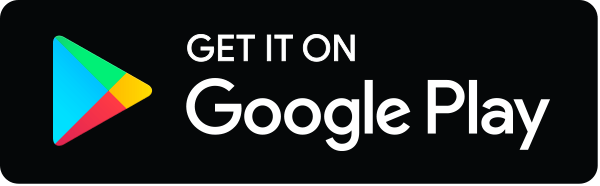