Abstract
Radiation therapy is commonly used in early-stage breast cancer treatment but must be delivered carefully to minimize the risks to critical adjacent structures, such as the heart and lung. Technological advances in radiation therapy have provided us with various methods for decreasing heart and lung doses, diminishing overall treatment time, and decreasing acute and long-term toxicity with more homogenous dose distributions. These technological advances in radiation therapy continue to evolve with even faster and more novel delivery techniques intended to further tailor the treatment to patients’ anatomy, improving patient convenience, and ultimately increasing the therapeutic ratio.
Keywords
breast cancer, radiation therapy, radiotherapy technique, dosimetry, toxicity
Radiotherapy Techniques: Introduction
Radiation therapy (RT) is one of the major therapeutic modalities used in the management of early-stage breast cancer and offers significant reductions in local-regional relapse after breast conserving surgery for most patients, as well as a potential for reducing breast cancer–specific mortality risk in selected subgroups. Breast conservation therapy (B), defined as local excision of the primary tumor followed by RT, is considered a standard management option for women with early-stage disease, based on multiple randomized prospective trials of invasive and noninvasive cancers with long-term follow-up. In the setting of invasive cancers, RT has been shown to reduce in-breast recurrences by a relative risk reduction of approximately two-thirds and also confers a small, but statistically significant, long-term survival benefit when delivered after breast conserving surgery. Similarly, RT has been demonstrated to reduce both invasive and noninvasive in-breast recurrences by approximately 50% in all subgroups across the wide spectrum of ductal carcinoma in situ (DCIS) subtypes, although the pooled data from meta-analysis fail to show a similar survival benefit with radiation for DCIS. In these early randomized trials that established the integral role of radiation as a component of B, the majority of patients received conventionally fractionated, whole breast radiation therapy (WBRT), delivered to doses of 45 to 50 Gy in 1.8- to 2.0-Gy fractions, over a 5- to 6-week period. Recent technological advancements have resulted in improvements in the therapeutic ratio of RT by minimizing radiation exposure to normal tissue (such as heart, lung, and unaffected breast) while maintaining tumor control and, in some cases, allow for more accelerated treatment delivery in selected patients, with options such as accelerated partial breast irradiation (APBI) and hypofractionated WBRT (hWBRT). This chapter reviews basic rationale and radiobiology of RT and describes modern methods and techniques currently used early-stage breast cancer.
Radiation Therapy Modalities: External Beam Versus Brachytherapy
There are two major, yet very different, modalities for the delivery of RT: (1) external-beam RT (EBRT), in which radiation is generated outside the body and delivers high-energy photon or electron x-ray beams to the intended target (whole breast, lumpectomy cavity, etc.), and (2) brachytherapy, in which radioactive sources are placed inside of the patient in close proximity to the tumor and typically emit radiation of lower energy intended to treat only the volume of tissue in the immediate vicinity to the source. Brachytherapy is the less common delivery technique that mandates dedicated equipment, sources, room shielding, and specific expertise for handling and delivery; EBRT is more widespread and most commonly delivered using cobalt machines or linear accelerators (LINACS). Cobalt machines deliver photon energies of 1.2 and 1.3 MV and have the ability to adequately penetrate and treat most tumors sites that are not located deep within tissue. Several major disadvantages that limit the use of cobalt machines in clinical practice include a larger penumbra (less precisely defined beam edge), inability to deliver adequate doses to deeper tissues, and exposure to radiation to therapy staff from radioactivity from the active Co-60 source. Many of the original RT phase III trials that initially established the benefits of RT used cobalt machines and subjected the patients randomized to radiation in these trials with inadvertent radiation exposure to normal tissues such as heart, lung, and contralateral breast. With long-term follow-up, there is a large body of data demonstrating the subsequent and significant consequences of older delivery techniques including higher risks of second malignancy (in lung and contralateral breast) and, in cases of left breast treatment, development of cardiovascular disease and cardiac-related deaths. Hence, cobalt machines across North America and Europe have mostly been replaced by LINACS in the past 25 years. Unlike Cobalt machines, LINACS do not continuously emit radioactivity so personnel exposure concerns are minimized. Furthermore, additional benefits include, but are not limited to, a wide range of beam energies extending from 4- to 6-MV photons (typically used for breast cancer) to 18 to 20 MV (sometimes used in larger breasted patients but more typically used for deep tumor sites such as those within the abdomen or pelvis). LINACs also offer a range of therapeutic electron beams, which are less penetrating and can be used in combination with photons. Contemporary LINACS also offer automated multileaf collimation, intensity-modulation capabilities, on-board imaging, and other technological advances all designed to deliver a more precise, conformal dose of radiation.
Alternatively, brachytherapy has been used to deliver an additional RT “boost” to the lumpectomy cavity after WBRT, for accelerated partial breast techniques (APBI, discussed later in the chapter), and for previously radiated patients who require retreatment to limited areas such as in the instance of chest wall recurrences. Brachytherapy can be delivered in conjunction with EBRT or alone but is generally not used to implant larger volumes or deeply situated tumor sites such as regional lymph nodes. The brachytherapy procedure typically requires the placement of radioactive sources into temporary catheter(s), which are placed in close proximity to the tumor or tumor bed. Iridium-192 (Ir 192 ) is the most commonly used source, has a half-life of 74.2 days, and emits an average photon energy of 0.38 MV, which allows for feasible shielding against radiation exposure for personnel. Modifications to the rate and distribution of RT into the surrounding tissues can be achieved by varying the type of implant catheters, the number of sources, and their placement. Hence, brachytherapy can be delivered as either “low-dose rate” (LDR) or “high-dose rate” (HDR) (see the ABPI section).
The beam characteristics of electron EBRT, high-energy photon EBRT, and brachytherapy (lower-energy) are important for understanding their application in breast cancer treatment. Generally, the higher the photon energy, the greater the penetration to deeper depths, but the lower RT dose to the skin surface. Electron beams travel a finite depth in tissue, therefore are useful for the RT “boost” dose, where an “en-face” electron field typically delivers RT to a limited area surrounding the lumpectomy cavity after WBRT, or for treatment of the chest wall or internal mammary nodes (for which more superficial treatment is advantageous to reduce exposure to underlying heart and lung). Electron beams similarly increase the depth of penetration with increasing energy; however, unlike photons, surface dose increases with increasing electron energy. The use of various photon or electron beam energies allows for tailoring the radiation dose distribution to the individual patient’s anatomy. Alternatively, the low-energy photons emitted from brachytherapy sources (Ir 192 ) do not penetrate tissues deeply and hence only deliver RT to a limited area surrounding the source.
Radiobiological Considerations
The rationale for using conventional fractionation (small daily fractions of 1.8–2.0 Gy per day to a total dose of 45–50 Gy) is based on theoretic radiobiological modeling of the relative sensitivity of normal tissue compared with cancer cells to changes in daily fraction size. Historically, normal tissue, relative to most tumor types, was considered to be more sensitive to fraction size. Thus smaller daily fractions have traditionally been used with the rationale that larger daily fractions theoretically increased the risk of damage to normal cells relative to tumor kill, resulting in a higher potential for late-reacting (normal) tissue complications. Breast cancers, like most other tumors, were presumed to have low sensitivity to changes in fraction size relative to normal tissue. More recently, it was established that for breast cancer explicitly, the sensitivity to fraction size is similar to that of surrounding normal tissue, based on radiobiologic calculations of cell survival curves of breast cancer cells relative to normal breast tissue. For example, for most tumor subtypes, delivering a 2-Gy fraction would result in more tumor kill compared with normal tissue kill, but if fraction size is increased to 4 Gy, normal cell kill becomes increasingly greater than tumor cell kill, resulting in a decrease in the therapeutic ratio (increasing toxicity relative to tumor cell kill). Unique to breast cancer, however, the cell survival curves of breast tumor cells relative to normal breast tissue are similar, suggesting similar sensitivity to changes in fractionation. Therefore, if increasing the fraction size has minimal effect on the therapeutic ratio in breast cancer, there may be little or no therapeutic advantage to using smaller daily fraction sizes in breast cancer. In fact, these radiobiological estimations are clinically supported by several phase III trials, which randomized patients to conventional fractionation compared with various hWBRT regimens, and suggest similar tumor control and noninferiority in late tissue complications and cosmesis. As such, hWBRT is now more routinely incorporated into clinical practice in selected subsets of patients, and has decreased the treatment delivery of EBRT from typically 5+ weeks to 3+ weeks. The vast majority of patients on these trials were treated to the intact breast (not postmastectomy), did not receive regional-nodal radiation or prior chemotherapy, and had invasive cancers, but the indications for appropriate patient populations for hWBRT are evolving.
Anatomic Considerations for Radiation Delivery
As the field of radiation oncology has moved toward more conformal techniques for delivering uniform doses of radiation throughout the breast and chest wall, reconstructed breast or chest wall and nodal target delineation is critical for accurate treatment delivery and better sparing of normal tissue. Significant interobserver variations in target delineation in breast cancer radiotherapy planning have been previously reported. Notably, the Radiation Therapy Oncology Group (RTOG) evaluated interobserver variability of target and normal structure delineation of nine radiation oncologists specializing in breast radiotherapy and found as little as 10% overlap, with standard deviations up to 60% in volume variation, for contouring of regional nodes and anatomic structures across this group of breast experts. To promote standardization of target volumes and improve quality, the RTOG created a breast contouring guidelines with accompanying atlas. The consensus guidelines are summarized in Tables 46.1 and 46.2 . Using predetermined anatomic definitions, an averaged contour was generated from this cohort of experts and confirmed using consensus software tools. These standards of volume definition should allow for future studies pertinent to radiation therapy techniques and/or treatment volumes to be more consistently report and improve upon reproducibility and generalizability.
Cranial | Caudal | Anterior | Posterior | Lateral | Medial | |
---|---|---|---|---|---|---|
Breast | Clinical reference + second rib insertion | Clinical reference + loss of CT-apparent breast | Skin | Excludes pectoralis muscles, chest wall muscles, ribs | Clinical reference + mid axillary line typically, excludes latissimus dorsi muscle | Sternal-rib junction |
Breast + chest wall | Same | Same | Same | Includes pectoralis muscles, chest wall muscles, ribs | Same | Same |
Chest wall | Caudal border of the clavicle head | Clinical reference + loss of CT-apparent contralateral breast | Skin | Rib-pleural interface (includes pectoralis muscles, chest wall muscles, ribs) | Clinical reference/mid axillary line typically, excludes latissimus dorsi muscle | Sternal-rib junction |
Cranial | Caudal | Anterior | Posterior | Lateral | Medial | |
---|---|---|---|---|---|---|
Supraclavicular | Caudal to cricoid cartilage | Junction of brachiocephalic-axillary veins/ caudal edge of clavicle head | Sternocleidomastoid (SCM) muscle | Anterior aspect of the scalene muscle | Cranial: lateral edge of SCM muscle Caudal: junction of first rib-clavicle | Excludes thyroid and trachea |
Axilla Level I | Axillary vessels cross lateral edge of pectoralis minor | Pectoralis major muscle insert into ribs | Plane defined by anterior surface of pectoralis major and latissimus dorsi muscle | Anterior surface of subscapularis muscle | Medial border of latissimus dorsi muscle | Lateral border of pectoralis minor muscle |
Axilla Level II | Axillary vessels cross medial edge of pectoralis minor muscle | Axillary vessels cross lateral edge of pectoralis minor | Anterior surface of pectoralis minor muscle | Ribs and intercostal muscles | Lateral border of pectoralis minor muscle | Medial border of pectoralis minor muscle |
Axilla Level III | Pectoralis minor muscle insert on cricoid | Axillary vessels cross medial edge of pectoralis minor | Posterior surface of pectoralis major muscle | Ribs and intercostal muscles | Medial border of pectoralis minor muscle | Thoracic inlet |
Internal mammary | Superior aspect of the medial first rib | Cranial aspect of the fourth rib | Encompass the internal mammary/thoracic vessels | Encompass the internal mammary/thoracic vessels | Encompass the internal mammary/thoracic vessels | Encompass the internal mammary/thoracic vessels |
External-Beam Techniques and Considerations
Standard Treatment Field Setup
The majority of patients who receive external beam radiotherapy to the intact breast are treated using tangential photon fields. Initially the patient undergoes a “simulation,” a procedure to obtain anatomic information that allows the design of the treatment fields. This can be done using a conventional x-ray simulator, which has the same geometry as the treatment machine but provides diagnostic-quality fluoroscopy and x-ray films, or, more recently, with a computed tomography (CT) simulator, which provides axial slices and more anatomic information to individualize dose distribution of the radiation beams. In either case, the patient is placed in the treatment position, typically supine with her arms positioned over her head to move the arm out of the radiation field and reduce the skin fold in the inframammary region. The typical borders of the chest wall/breast radiation fields are superiorly the inferior clavicular head; inferiorly 2 to 3 cm below the inframammary fold; medially the midsternum; and laterally 2 cm beyond palpable breast tissue (often at the anterior border of the latissimus dorsi muscle). At the time of “simulation,” adhesive wires can be placed to encompass the clinical breast volume, as well as to highlight the lumpectomy scar, which are useful to visualize and delineate the clinical breast volume on the imaging with either fluoroscopy or CT images. These images are ultimately used to generate a radiation treatment plan customized for each individual patient’s anatomy.
Two-Dimensional Versus Three-Dimensional Conformal Versus Intensity-Modulated Radiation Therapy
Historically, breast radiotherapy treatment planning consisted of two-dimensional planning, which consisted of fluoroscopic simulation to establish treatment fields. The radiation beams were arranged tangentially to limit the exposure to underlying heart, lung, and contralateral breast, but the dose calculations were based on treatment planning using only a single plane in the middle of the breast (central axis). However, given the variations in the contour of breast tissue from its superior to inferior extent, there was significant heterogeneity of the dose distribution across the treatment plan, leading to “hot spots” and or “cold spots” that were not accounted for (i.e., distribution of dose within the breast will be greatest in the thinnest portions of the breast leading to “hot spots” and vice versa). In the modern era of radiotherapy, the use of CT scanners has allowed for three-dimensional (3D) planning to replace earlier two-dimensional (2D)-based planning methods and better define target volumes across the entire breast volume (not just at the central axis) and more precisely avoid organs at risk. Increasingly sophisticated algorithms use 3D information to conform the dose distribution, to the target and provide more homogenous doses of radiation treatment.
Several methods are available to deliver a more homogeneous dose to the breast/chest wall volume. MLC takes advantage of the small computer-controlled motorized small metallic leaves in the head of the machine, which essentially function as automated mini blocks to deliver a more conformal dose by shaping the radiation beam edges. Furthermore, “wedges,” are often used, which are wedge-shaped pieces of metal used to attenuate the beam depending on the slope and thickness of the breast or chest wall to achieve a more uniform distribution of the radiation dose by attenuating the dose delivered the breast/chest wall to better match its contour. When electron beams are used, such as for internal mammary nodal treatment or lumpectomy “boost dose,” physical blocks are placed in the path of beam in the machine head to deliver a more conformal electron beam dose. Three-dimensional treatment techniques make use of subfields within the larger fields (field in field) to decrease the “hotspots,” achieved using the MLCs described earlier. Dose inhomogeneity is associated with worse skin toxicity, breast pain, and poorer long-term cosmesis ; therefore these methods are routinely used to improve radiation dose homogeneity. Unlike 2D treatment planning, 3D planning requires evaluation of the dose distribution over the entire breast, not just at the central axis. The field-in-field techniques can be forward or inverse planned, and use “subfields” that block segments of tissue that would otherwise be receiving higher doses ( Fig. 46.1 ). Modifications to the weighting of these subfields and mixing of photon energies can optimize dose homogeneity.
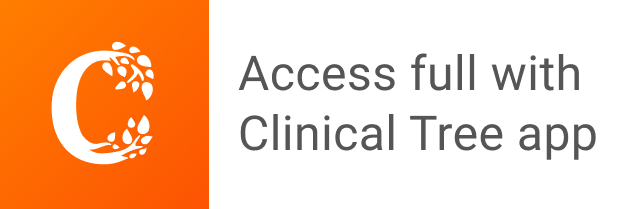