How T Cells Recognize Antigen: The Role of the Major Histocompatibility Complex
Introduction
Previous chapters have focused on the development and function of B cells, their antigen-specific receptors (immunoglobulin [Ig]), and the mechanisms by which B cells generate a huge array of clonally distributed receptors. Antibodies, the antigen-specific products of B cells, play a critical role in the interaction with antigens outside cells, such as viruses or bacteria encountered in blood or at mucosal surfaces. However, many pathogens—particularly viruses, parasites, and some bacteria—invade host cells and live at least part of their life cycle inside them. Antibodies do not enter cells, so once a pathogen gains entry to a host cell, antibodies are ineffective at defending the host. The immune response to pathogens inside host cells is the domain of T cells and their products. T cells also mount responses to “harmless” antigens—foreign agents that are not pathogenic but to which we need to respond and eliminate.
Antibodies bind to all types of antigens, regardless of whether the antigens are protein, carbohydrate, nucleic acid, or lipid. By contrast, T cells respond almost exclusively to proteins, or more precisely, small peptides derived from the catabolism of proteins. (Later in the chapter we describe additional types of antigens that some subsets of T cells respond to.) Proteins are major constituents of pathogens and are also the products of viral infection. In addition, most other antigens are protein in nature. Thus, T cells play a critical role in the response to nearly all potentially harmful agents and the myriad of other antigens to which an individual is exposed.
Because T cells deal with pathogens and antigens that infect or are taken into host cells, they use an antigen recognition system distinct from the one used by B cells: T cells interact with antigens expressed on the surface of host cells. However, like B cells, T cells express an antigen-specific receptor, the T-cell receptor (TCR).
Before we discuss the properties of the TCR (Chapter 10), in this chapter we describe how T cells recognize antigen, and the role played by major histocompatibility complex (MHC) molecules in this recognition. We will focus on antigen recognition by the major set of T cells, known as αβ T cells, which express the two-chain molecule αβ as their TCR. Unless otherwise indicated we will refer to these cells as simply “T cells.” Later in the chapter we discuss antigen recognition by other subpopulations of T cells. We will also discuss the characteristics of the genes that code for MHC molecules, the enormous and unique diversity of these molecules within the population, and possible reasons for this diversity.
How The MHC Got Its Name
The term major histocompatibility complex derives from research in transplantation that started in the mid-twentieth century. These experiments provided insight into the rules governing the acceptance or rejection of tissues—literally, histocompatibility—when tissues were transplanted between different members of the same species (generally mice; see Chapter 19 for more discussion). Researchers interpreted their early findings to indicate that rapid rejection of such transplants was determined by a single gene, which they called the major histocompatibility gene. Because later studies indicated that this “gene” was in fact a complex—a set of closely linked genes inherited as a unit—it became known as the major histocompatibility complex. We know now that every vertebrate species has an MHC containing multiple genes. The human MHC is known as HLA (human leukocyte antigen).
Other early studies of transplantation in mice indicated that T cells played an important role in the rejection response (see Chapter 19 for more details). Taken together, these transplantation studies demonstrated an important but not well-understood connection between the MHC and T-cell responses. Because individuals do not normally undergo transplants, the function of the MHC in “everyday” T-cell responses became the focus of intense investigation.
The everyday function of the MHC is described in the sections that follow.
MHC Role in Antigen Presentation
The events that occur inside a host cell after a protein antigen has entered it are summarized in Figure 9.1.
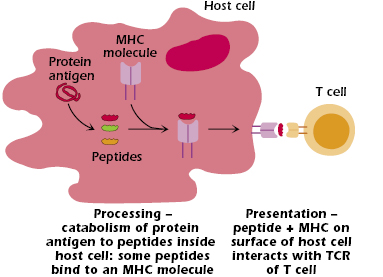
In summary:
- The protein is broken down (catabolized or “processed”) to peptides—linear fragments—of varying length.
- Some of these peptides bind to an MHC molecule inside the cell. This binding is selective; that is, not all the peptides formed bind to MHC molecules.
- The MHC molecule with bound peptide moves to the cell surface.
- The combination of peptide bound to an MHC molecule is recognized at the cell surface by a T cell that expresses the “appropriate” or “correct” TCR—one of the billions of different TCRs the host can generate. Figure 9.2 shows the three critical components of T-cell recognition of antigen: peptide, an MHC molecule expressed on the surface of a host cell, and the TCR expressed on a T cell.
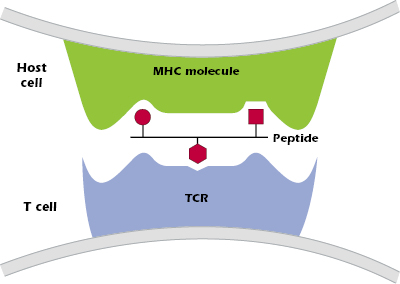
Thus, MHC molecules have two key functions: (1) to selectively bind to peptides produced when proteins are processed inside cells of the host, and (2) to present peptides on the surface of a host cell to a T cell with the appropriate TCR.
The critical role played by MHC molecules in binding processed antigen and presenting it in T-cell responses is referred to as the MHC restriction of T-cell responses.
Multiple copies of each MHC molecule are expressed on the surface of a host cell, and each MHC molecule can bind many peptides (one peptide at a time, as we explain later in the chapter). By binding to peptides inside the cell, MHC molecules “sample” the internal environment of host cells and present information on the cell surface that allows T cells to identify whether a particular host cell has been infected, or contains some foreign component. The combination of MHC molecule plus foreign peptide expressed on the surface of a host cell is a key signal to host T cells that they need to respond. An important corollary is that our T cells do not respond to host cells in the absence of foreign peptide: our T cells focus on responding to infected cells (or cells that contain antigen) but do not respond to host cells that are uninfected.
MHC molecules also play a key role during the differentiation of T cells in the thymus (see Chapter 10). Thus, MHC molecules play important interrelated roles in both the differentiation of immature T cells and in the responses of mature T cells.
Different MHC Molecules Are Expressed by Distinct Host Cells and Interact with Different Sets of T Cells
Two major sets of MHC molecules—MHC class I and MHC class II molecules—direct distinct T cell responses (Figure 9.3).
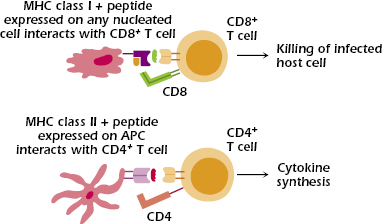
MHC Class I
MHC class I molecules interact with CD8, whose expression defines the subset of T cells called CD8+ T cells. Thus, to expand on the definition of MHC restriction of T-cell responses we introduced earlier, we say that the responses of CD8+ T cells are restricted by MHC class I molecules.
MHC class I molecules are expressed on all nucleated cells (thus, not on red blood cells), any of which may be infected by a pathogen such as a virus, bacterium, or parasite. The main function of CD8+ T cells is to kill pathogen-infected host cells, as well as tumors and transplanted tissue. Thus, MHC class I molecules and CD8+ T cells play critical roles in the responses to pathogens that infect host cells.
In addition to their interaction with CD8 expressed on CD8+ T cells, MHC class I molecules also interact with molecules expressed on natural killer (NK) cells. This interaction prevents NK cells from killing normal host cells.
MHC Class II
MHC class II molecules interact with CD4, whose expression defines the subset of T cells called CD4+ T cells. In parallel with our definition in the previous section, we say that the responses of CD4+ T cells are restricted by MHC class II molecules.
MHC class II molecules have a more limited distribution than MHC class I molecules: They are expressed constitutively (that is, under baseline conditions) only on antigen-presenting cells (APCs) but can be induced on other cell types. APCs are cells that take up antigen and present it to T cells. In humans, the principal APCs that express MHC class II are dendritic cells, macrophages, and B lymphocytes; thymic epithelial cells (discussed in the next chapter) also express MHC class II molecules. In the absence of inducing factors, most cells (for example, liver and kidney tissue cells) express MHC class I but not MHC class II molecules; by contrast, APCs constitutively express both MHC class I and class II molecules.
In response to activation, CD4+ T cells synthesize a vast array of cytokines, and hence cooperate with multiple types of cells, including helping B cells synthesize antibody. Thus, MHC class II molecules and CD4+ T cells play critical roles in the responses to agents—pathogens and antigens—that are taken into APCs.
The expression of both MHC class I and II molecules can be affected by many factors. Cytokines released during the response to infectious agents enhance the expression of MHC molecules: Interferon (IFN) α, β, and γ upregulate MHC class I expression, and IFN-γ upregulates MHC class II expression. As a consequence of this upregulation, MHC class II expression is induced on cells such as fibroblasts and endothelial cells that do not normally express it, and increased on APCs. Induction and increased expression of MHC class I and II molecules thus enhance T-cell responses to infectious agents. On the other hand, some virus infections and tumor development result in decreased expression of MHC molecules. We will discuss the regulation of expression of MHC class molecules in greater detail later in this chapter.
Variability of MHC Class I and MHC Class II Molecules
Before discussing the structures of MHC molecules, we note an important point: MHC class I and class II molecules differ from individual to individual within the population, and these differences are genetically determined; that is, MHC-distinct individuals express MHC molecules with somewhat different sequences.
These differences in MHC molecules from individual to individual arise from two sources: polygenicity and polymorphism. Polygenicity means that MHC class I and II molecules are coded for by multiple independent genes. The human HLA complex (found on chromosome 6) contains three independent genes—HLA-A, HLA-B, and HLA-C—that code for MHC class I molecules (Figure 9.4).
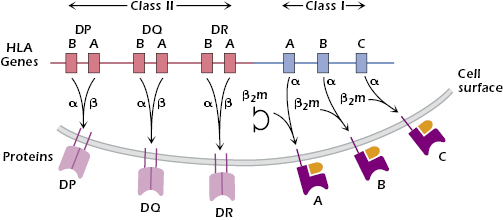
As we explain below, each HLA class I molecule is expressed at the surface in association with a small molecule, β2-microglobulin (β2m), coded for outside the HLA complex. Because every cell has two sets of chromosomes (one paternally derived and one maternally derived), every nucleated cell may express up to six different HLA class I molecules, each capable of binding peptides. Similarly, the HLA complex codes for three different two-chain MHC class II molecules: HLA-DP, HLA-DQ, and HLA-DR (Figure 9.4). Thus, human APCs may express up to six different HLA class II molecules, each capable of binding to peptides.
Polymorphism means that multiple stable forms of each MHC gene exist in the population. The MHC is the most highly polymorphic gene system in the body and hence in the population: In humans over a thousand slightly different versions—alleles—of the gene that codes for the MHC class I molecule HLA-B and MHC class II molecule HLA-DRB have been identified. Other important examples of genetic polymorphism in humans are the different forms of the red blood cell antigens (A, B, and O) and of hemoglobin molecules. Recent studies comparing gene sequences from different individuals—by identifying single nucleotide polymorphisms—have found that many genes show allelic variation, for example, genes coding for cytokine receptors and liver detoxification enzymes. However, none of these genes is as variable within the population as HLA.
The extensive polymorphism of human MHC genes makes it very unlikely that two random individuals will express identical sets of HLA class I and class II molecules. As we describe in Chapter 19, the enormous diversity of MHC molecules and the genes that code for them is a major barrier to successful transplantation of organs and tissues. Later in this chapter we discuss why we believe the MHC evolved to be so diverse.
Note that the mechanisms used to generate the diversity of MHC structures differ from the mechanisms used to generate the diversity of the antigen-specific receptors of B and T cells (Ig and TCR, respectively) that arises from rearrangement of DNA and which produces one type of receptor per cell (Chapters 7 and 10). In contrast, although MHC molecules are diverse within the population, each cell in a particular individual (liver, kidney, lymphocytes, etc.) expresses the same set of HLA class I and class II molecules.
Structure of MHC Class I and Class II Molecules
In the sections below we refer to “typical” MHC class I and II molecules to illustrate the general features of their structure. These “typical” features mean they are common to humans and other vertebrate species (for example, mice, cats, and cattle).
MHC Class I
Parts A–D of Figure 9.5 show different ways to represent the key features of the structure of a typical MHC class I molecule and how it interacts with peptide and a TCR. An MHC class I molecule is a transmembrane glycoprotein (molecular weight approximately 43 kDa), expressed on the cell surface in noncovalent association with a small invariant (identical on all cells) polypeptide called β2-microglobulin (β2m; molecular weight 12 kDa). As we noted above, β2m is encoded by a gene on a separate chromosome from the MHC. The MHC class I molecule is referred to as the α or heavy chain and comprises three extracellular Ig-like domains—α1, α2, and α3. β2m has a structure homologous to a single Ig domain; indeed, β2m and MHC class I are members of the Ig superfamily described in Chapter 5. At the cell surface, MHC class I plus β2m has the appearance of a four-domain molecule—α1 paired with α2 on the exterior of the MHC class I molecule and α3 and β2m paired closer to the membrane.
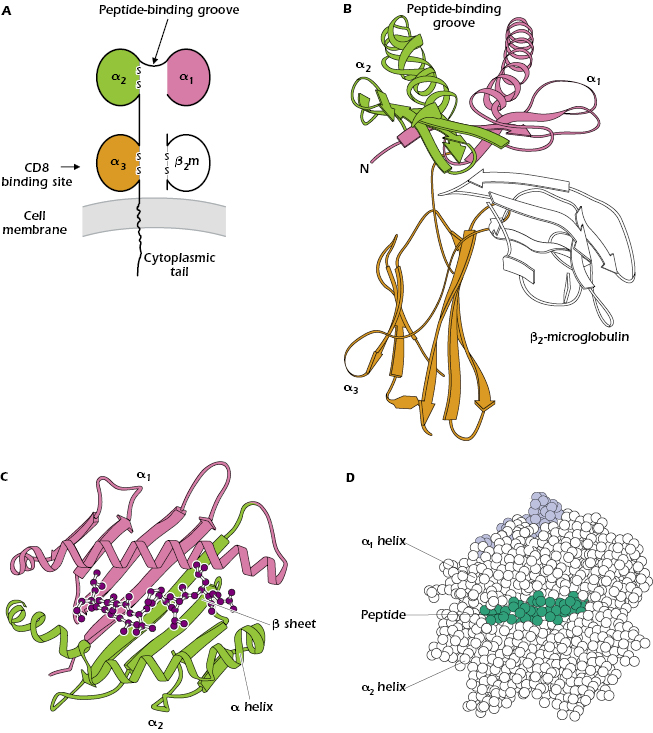
Figure 9.5B shows the most striking feature of all MHC class I molecules that have been examined by X-ray crystallography: a deep groove or cleft—the peptide-binding groove—in the part of the molecule farthest from the membrane that is composed of parts of the α1 and α2 domains. This groove can hold one peptide 8–9 amino acids in length in a linear array (see also Figure 4.5 of Chapter 4). Every MHC class I molecule can bind several different peptides, but only one at a time. Figure 9.5C shows that the groove resembles a basket with an irregular floor (made up of amino acids in a β-pleated sheet structure) surrounded by walls (formed by α helices). The bound peptide fits inside the groove, as illustrated in both Figures 9.5C and 9.5D.
Selectivity of Peptide Binding to MHC Class I Molecules.
We referred above to the tremendous variability of MHC class I molecules within the population. Looking at differences in sequence among MHC class I molecules, we find that most of the differences in amino acids are confined to a limited region in the extracellular α1 and α2 domains, and particularly in the floor and walls of the peptide-binding groove (see Figure 9.5C). These differences in amino acid sequence and hence structure of the binding groove play a critical role in determining which peptides bind to a particular MHC molecule. The pockets forming the floor of the groove also help to align peptides so they can be recognized by specific TCRs (Figure 9.6).
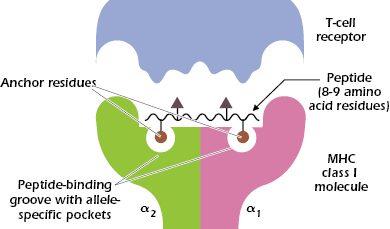
Thus, binding of peptide to an MHC class I molecule is selective: One MHC molecule will bind with high affinity to only certain peptides. A single MHC class I molecule preferentially binds peptides with specific anchor residues: invariant or closely related amino acids at certain positions in the 8- or 9-amino-acid sequence. As shown in Figure 9.6, a peptide that binds to an MHC class I molecule typically has two anchor residues, which interact with the allele-specific pockets in the MHC molecule. The other positions in the peptide may vary. As a result, one MHC molecule can bind a large number of peptides with different sequences. This helps explain how only a maximum of six MHC class I molecules in an individual can display many different peptide antigens. It also helps explain why with very few exceptions T-cell responses are made to at least one epitope from almost all proteins and why failure to respond to a protein antigen is so rare.
Figure 9.6 also shows that the peptide bound in this cleft and parts of the MHC class I molecule interact with the TCR. One to four amino acids in the peptide make contact with the TCR, indicating that only a small number of contacts with the peptide are critical for recognition by the TCR.
CD8 Binding to Invariant Region of MHC Class I Molecules.
Outside the peptide-binding cleft the sequences of different MHC class I molecules are very similar. Thus an individual MHC class I molecule can be divided into a polymorphic or variable region (sequence unique to that molecule) in the area in and around the peptide-binding groove, and a nonpolymorphic or invariant region that is similar in all MHC class I molecules. CD8, the molecule that characterizes the CD8+ T-cell subset, binds to the invariant region of all MHC class I molecules, specifically in the α3 domain (see Figure 9.5A).
Structure of MHC Class II Molecules
Figure 9.7 shows different ways to represent the key features of a typical MHC class II molecule. Figure 9.7A shows that an MHC class II molecule is a transmembrane glycoprotein comprising two chains: α and β (molecular weight of approximately 35,000 and 28,000 Da, respectively). Like MHC class I molecules, every MHC class II molecule is expressed at the cell surface as a four-domain structure: the α1 domain is paired with β1, and α2 with β2. The chains α and β have cytoplasmic tails and extracellular Ig-like domains; they are also members of the Ig superfamily.
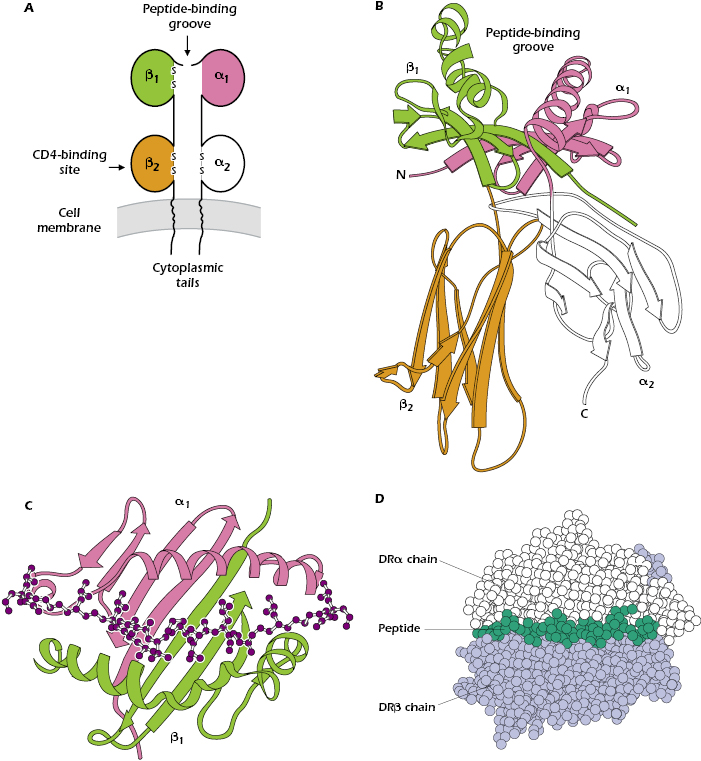
Like the MHC class I molecule, the MHC class II molecule contains a peptide-binding groove at the top of the molecule (shown in more detail in Figures 9.7B and 9.7C), which holds one peptide. However, in the MHC class II molecule, the groove is formed by interactions between the α1 and β1 domains. Figure 9.7C indicates that the floor and walls of the MHC class II cleft have the same β-pleated sheet and α-helical structures found in the MHC class I molecule.
In contrast to the 8- to 9-amino-acid peptides that bind to the cleft in the MHC class I molecule, the MHC class II groove binds peptides varying in length from 12 to approximately 17 linearly arranged amino acids. Figures 9.7C and 9.7D show that the ends of the peptide are outside the peptide-binding groove.
As with MHC class I molecules, each MHC class II molecule binds to peptides with specific anchor residues. Figure 9.8 shows that a peptide that binds to a typical MHC class II molecule has three (sometimes four) anchor residues in the central region of the peptide that bind to the allele-specific pockets of the MHC class II molecule. Because the other amino acids in the peptide outside the anchor residues may vary, MHC class II molecules are also capable of binding a wide selection of peptides. Between four and six of the peptide’s amino acids contact the TCRs; only two are shown in Figure 9.8.
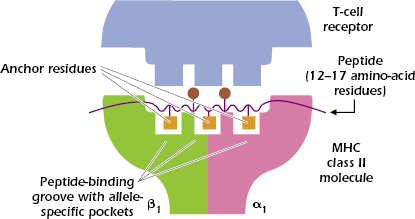
Like MHC class I molecules, MHC class II molecules are composed of variable or polymorphic regions, and invariant or nonpolymorphic regions. CD4, the molecule that characterizes the CD4+ T-cell subset, binds to the invariant region of all MHC class II molecules, specifically in the β2 domain (see Figure 9.7A).
Antigen Processing and Presentation: How MHC Molecules Bind Peptides and Create Ligands That Interact with T Cells
Exogenous Antigens and Generation of MHC Class II–Peptide Complexes
As the term implies, exogenous antigens
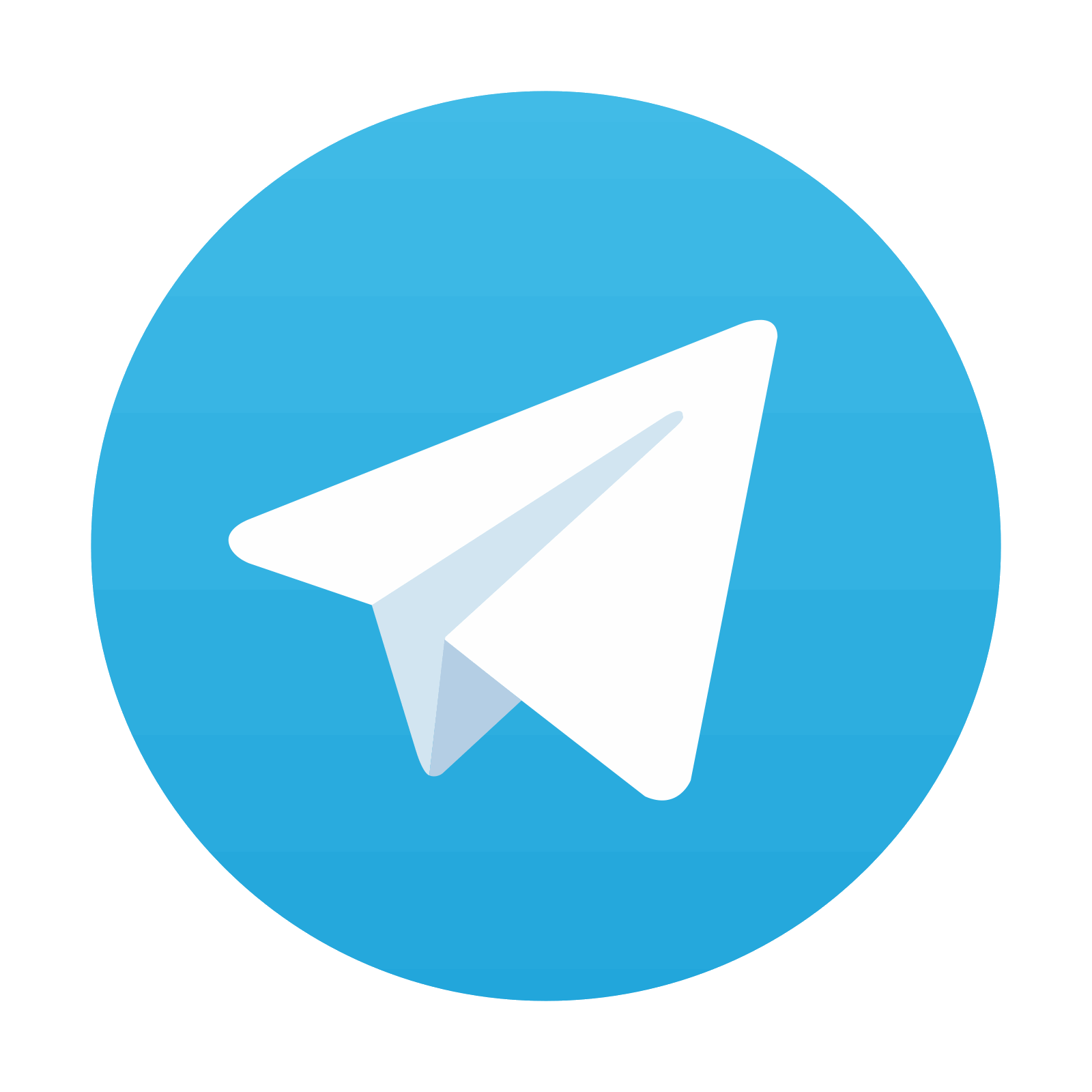
Stay updated, free articles. Join our Telegram channel
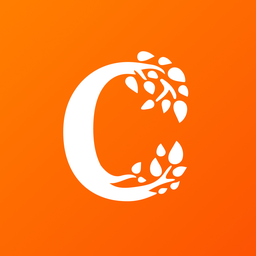
Full access? Get Clinical Tree
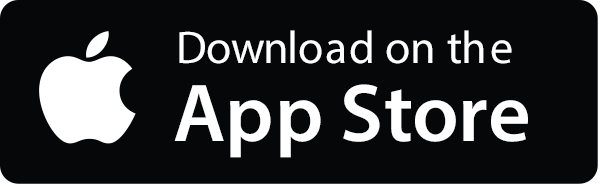
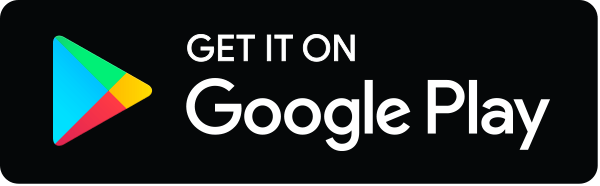