- Nutrition and fluid/electrolyte balance are closely linked.
- Starvation, injury, or surgical stress can lead to disturbed fluid and electrolyte balance.
- Fluid can shift to the interstitial compartment during starvation, injury, or surgical stress and lead to tissue oedema.
- The regulation of fluid within body water compartments is achieved through the control of ECF osmolarity.
- Thirst is a behavioral response to loss of body fluid. Together with reflex endocrine and neural responses, thirst is responsible for maintaining the homeostasis of body fluids, and more specifically for the regulation of the ECF compartment.
- Starvation, severe trauma, sepsis, and other disease processes can cause alterations in the sizes of the body water compartments and in exchangeable ions, and may acutely alter the volume and composition of the ICF.
- Intravenous fluid therapy can cause morbidity when associated with fluid overload. Harmful effects on the cardiorespiratory, gastrointesinal, and renal organ systems can be avoided with a balanced approach to supplementary fluid volume and composition.
3.1 Introduction
Starvation affects a number of metabolic processes central to homeostasis and these effects may be exacerbated by the presence of critical illness and surgical or traumatic stress. Furthermore, the control of metabolism and that of fluid/electrolyte balance are closely linked. In normal circumstances, the absorption of fluid and nutrients from the gastrointestinal tract counters the losses incurred during metabolism and cellular respiration. As such, the disruption of homeostatic mechanisms during starvation or injury has consequences not only for nutritional status but for the regulation of fluid and electrolytes. In this chapter, the principles of water/electrolyte balance and their impact on metabolic processes relevant to clinical nutrition will be discussed.
3.2 Fluid compartments of the body
As total body water makes up about 60% of body weight, an appreciation of the body water compartments (Figure 3.1) and the processes by which they are maintained is vital for an understanding of water/electrolyte balance. The intracellular fluid (ICF) compartment accounts for ~40% of total body weight (TBW), with cells separated from the extracellular fluid (ECF) (~20% of TBW), in which they are bathed, by the cell membrane. The ECF is divided into two further compartments by the capillary membrane: intravascular fluid (~5% of TBW) and interstitial fluid, which is intercellular (~15% of TBW).
Although impermeable to most molecules and proteins, some substances, such as water, carbon dioxide, and oxygen, can diffuse freely across the cell membrane. In addition, the cell membrane houses transport mechanisms to facilitate the inward transport of nondiffusible nutrients required for cellular metabolism as well as the selective expulsion of molecules to maintain a stable internal environment. Central to this vital process is the Na+/K+-ATPase pump, which maintains intracellular concentrations of sodium and potassium at 8 and 150 mmol/l respectively, in contrast to the concentrations of 140 and 5 mmol/l typically found in the ECF. The electrolyte concentrations found in body water compartments are outlined in Table 3.1.
Figure 3.1 The body water compartments. Figures are approximate percentages of body weight in the average individual.
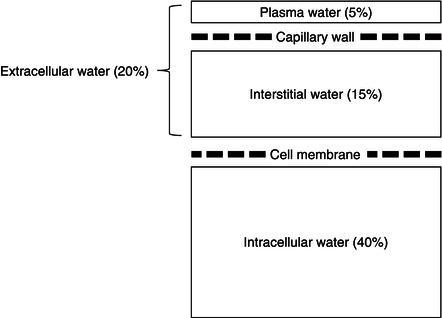
Table 3.1 Electrolyte concentrations in body water compartments. ECF, extracellular fluid; ICF, intracellular fluid.
The negative charges on intracellular protein molecules also help retain potassium within the cell (Gibbs–Donnan equilibrium). Correspondingly, shifts of water into or out of the cell are dependent on the osmotic gradient between the ECF and the ICF. With increased osmolarity of the ECF, water moves from the ICF to the ECF, resulting in cellular dehydration. Conversely, if salt is lost from or water added to the ECF to cause a hypotonic environment, water shifts from the ECF into the cell.
In blood vessels, the pores in the capillary membrane allow water, electrolytes, nutrients, and some macromolecules to cross from the circulation to the tissues. This movement of fluid across the capillary membrane is largely determined by the forces described by Starling’s equation:
where:
- ([Pc − Pi] − σ[πc − πi]) is the net driving force;
- Kf is the proportionality constant;
- Jν is the net fluid movement between compartments.
According to Starling’s equation, the movement of fluid depends on six variables:
Increased vascular hydrostatic pressure or decreased plasma oncotic pressure (Figure 3.2) facilitates the outward flow of water from blood vessels.
Figure 3.2 Diagrammatic model showing the effect of hydrostatic and oncotic pressure gradients on fluid movements across the capillary wall. P, hydrostatic pressure; Π, oncotic pressure; cap, capillary; int, interstitial.
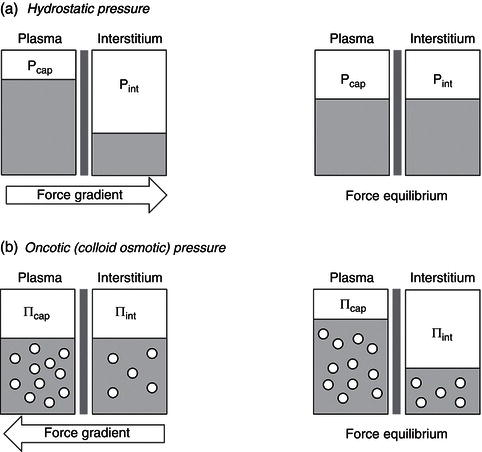
In tissues, the interstitial space is separated from the circulation by the capillary membrane and from the ICF by the cell membrane. The interstitial space acts as a buffer for changes which occur in plasma volume. Regulation of the interstitial volume is governed by local factors, which include Starling’s forces, but also the adequacy of lymphatic drainage, enabling fluid, and colloid molecules to be returned to the circulation. In health, there is continual leakage of albumin from the intravascular space, which occurs at a rate of about 5% per hour (Figure 3.3a). This rate can increase up to fourfold in critical illness, causing a shift of fluid towards the interstitium. Return of albumin to the circulation occurs via the lymphatic system, and as the rate of lymphatic drainage from the interstitium remains largely stable or is reduced by pressure generated by muscle contraction, excessive fluid transfer to the interstitium can cause tissue oedema (Figure 3.3b). This form of fluid transfer between the plasma and interstitium accounts for the rapid swelling of acutely inflamed tissues and the oedematous swelling characteristic of limb venous thrombosis.
Factors that influence the escape of plasma proteins from the intravascular space, thereby lowering the plasma oncotic pressure, include the size of membrane pores and properties of the endothelial glycocalyx – a complex structure comprising membrane-bound proteoglycans, glycoproteins, and bound plasma constituents. The endothelial glycocalyx forms a protective layer against the shear forces of blood cells on the luminal surface of the endothelium and has been shown to play an important role in barrier function, leukocyte adherence, and platelet aggregation. In capillaries, the layer is 0.5 µm thick; however, with greater vascular diameter, the glycocalyx layer increases in thickness. This thickness may be important as a determinant of vascular permeability and may become denuded with the interaction of drugs, enzymes, cytokines, or ischaemia and reperfusion, thereby reducing intravascular oncotic pressure and promoting tissue oedema.
The mechanism by which macromolecules escape from the intravascular space is described by the two-pore theory. In this model, small solutes are able to pass through small pores (radius: 2.5–3.0 nm) in the capillary membrane, present throughout the entire microvascular bed. Conversely, larger molecules can only pass through larger pores (radius: 10–11 nm) sited on the venous side of the capillary network. The small pores outnumber the large pores by 3000–3600 : 1. The transit of macromolecules across the pores occurs mainly by convection. Hence, an increase in capillary hydrostatic pressure caused by an expansion of plasma volume can result in leakage of larger molecules to the extravascular space, leading to the appearance of tissue oedema.
Figure 3.3 Capillary permeability and albumin flux in (a) health and (b) critical illness. ISS, interstitial space; IVS, intravascular space.
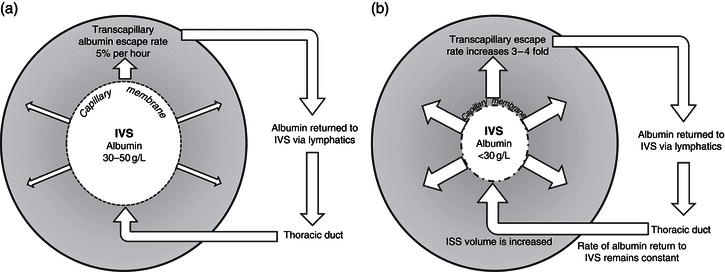
Further to the aforementioned compartments, it has been a traditionally held belief that a supplementary internal compartment, termed the ‘third space’, can arise in circumstances of trauma or blood loss. Fluid transfer to this compartment had been deemed distinct from the anatomical fluid shifts known to occur during illness, where fluid can accumulate within peritoneal or pleural cavities or the interstitial space of traumatised tissue but is ultimately subject to redistribution to the circulation. This model of fluid transfer to a proposed ‘third space’ has been the subject of controversy, with many highlighting the lack of firm evidence to support the concept. Since the anatomical location of the ‘third space’ has remained unclear, direct measurement of its volume has proved difficult, leading to questions over its exact nature.
Notwithstanding controversy over the existence of a ‘third space’, compartmentalisation is of major importance to maintaining the stability of the intracellular environment in the face of changes in both the intravascular and extracellular environments. It also allows the renal and gastrointestinal tracts to play an integral role in fluid and electrolyte balance, systems which effect responses to fluctuations in intra- and extravascular fluid composition. This is achieved through complex neuroendocrine interactions, which in due course promote the secretion or excretion of water with a different osmolality to that found in plasma.
3.3 Flux of fluid through the kidney and gastrointestinal tract
The kidneys and gastrointestinal tract play key roles in the maintenance of ECF volume and electrolyte balance in the face of variable daily fluid intake. This is important because mammalian tissues require a medium of fairly constant salt and water composition for normal function. Of equal importance, however, is the need to preserve enough fluid within the circulation to maintain blood pressure. It is clear that systemic blood pressure is dependent on the volume of blood within the vascular system and is a product of cardiac output and systemic vascular resistance. Cardiac output is related to the effective circulatory volume, and variations in these parameters normally parallel changes affecting the ECF volume. Accordingly, the regulation of blood pressure by the kidneys is accomplished by the control of ECF volume. This is an impressive feat when one considers that in a period of 24 hours, the two kidneys filter approximately 180 litres of ECF, with all but 1–2 litres of water and 50–100 mmol of sodium being reabsorbed. The volume of the ECF is influenced by its osmolarity and can be derived from the relationship:
Therefore, in regulating the osmolarity of the ECF, the kidneys are, in essence, able to regulate ECF volume and hence effective circulatory volume. In addition, the kidneys are able to detect changes in afferent arteriolar pressure using pressure detectors at the juxtaglomerular apparatus and subsequently to direct renal responses to the excretion or reabsorption of sodium and water through the renin–angiotensin–aldosterone system (RAAS). In this way, the kidneys are able to integrate information derived from both pressure and osmoreceptors to infer changes in blood volume status and execute the appropriate responses to modify ECF volume.
Cooperating with the kidneys in the control of fluid and electrolyte balance is the gastrointestinal tract, with 9 litres of fluid, containing a sodium load of 110–120 mmol/l, passing through the small bowel daily and only 150 ml being lost in stool (Figure 3.4).
Most of the fluid that passes through the gastrointestinal tract is derived from the secretions of the ECF rather than oral ingestion, and as such, small changes in absorptive capacity can have a profound effect on fluid and electrolyte balance. Nearly all of this fluid is reabsorbed from the gastrointestinal tract, facilitated by a Na+/K+-ATPase-generated osmotic gradient, as well as the co-transport of sodium with organic solutes, such as glucose, amino acids, or bile acids, in the small intestine and short-chain fatty acids in the colon.
In circumstances where there is deficit in effective circulatory volume, the reabsorption of both sodium and water from the gastrointestinal tract is enhanced. This is facilitated by the action of components of the RAAS on the small bowel mucosa, where receptors for aldosterone, angiotensin I, and angiotensin II are known to exist. In this way, the kidneys and gastrointestinal tract are able to integrate their roles in fluid and electrolyte balance.
3.4 Body electrolyte content and concentration
As mentioned previously, intravascular and ECF volumes are essentially preserved by the factors controlling body sodium, the predominant cation found within these compartments. For mammals, evolutionary pressures have led to the development of efficient mechanisms to preserve sodium and water, allowing survival in environments where these nutritional commodities might be scarce. On the other hand, there have been few evolutionary precedents demanding a sustained response to water and sodium excess. Thus, in the main, water- and sodium-retaining mechanisms are much more developed and powerful than those designed for water and electrolyte excretion.
Figure 3.5 Diagrammatic model showing the effect of the osmotic pressure gradient on water movement across the cell membrane.
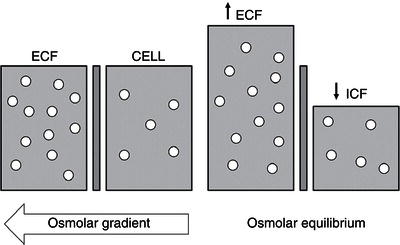
Total body sodium equates to approximately 3000–4000 mmol, with 53% of this available as an exchangeable fraction. The remaining 47% is considered part of a non-exchangeable fraction and exists within bone. Furthermore, most of the exchangeable fraction is located within the ECF, contrasting with an intracellular fraction of only 9%. Daily sodium intake varies, but on average amounts to 1 mmol/kg, and is equivalent to the amount excreted in the urine and faeces. In health, daily sodium losses are readily compensated for by the substantial reserves of the exchangeable fraction.
The body contains an equivalent amount of potassium as sodium, but in contrast to sodium the majority of the exchangeable fraction of potassium is intracellular. Furthermore, extracellular concentrations of potassium are under stringent control, as even small fluctuations can be clinically harmful. Any excess is usually excreted by the kidneys, but in critical illness, elevated potassium concentration within the ECF is compounded by the cellular efflux of amino acids and glucose, which are accompanied by potassium to maintain electrical neutrality.
Fluid shifts between ECF and ICF compartments
Water is able to move freely across most cell membranes, facilitated by transmembrane water channels known as aquaporins. Except for brief periods, the ECF and ICF remain in osmotic equilibrium. Hence, a measurement of plasma osmolarity provides a reasonable measure of both ECF and ICF osmolarity. On the other hand, the movement of ions across cell membranes depends on the presence of specific transporters. These transporters maintain a stable intracellular environment and counter minor fluctuations in ion concentration. In real terms, there is no appreciable inward shift of ions across the cell membrane. It can, therefore, be assumed that equilibration between ECF and ICF osmolarity occurs principally by the movement of water, and not by the movement of osmotically active solutes. In the main, these water shifts occur only when the ECF osmolarity is altered significantly (Figure 3.5).
3.5 Regulation of body water compartments
Physiological mechanisms to match the intake of water to output have evolved with the specific aims of maintaining extracellular osmolarity and protecting effective circulatory volume. These mechanisms detect changes in the volume of body water compartments and then signal the appropriate response in terms of excretion of sodium and water.
Coupled to these detectors are neuroendocrine pathways which promote the intake and retention of water from the kidneys and gastrointestinal tract, and complementary pathways, in order to promote the secretion and excretion of fluid when necessary. These pathways are discussed further in this section.
Control of body fluid osmolality
The ability to excrete urine with a different osmolality from plasma plays a central role in the regulation of water balance. When plasma osmolality is decreased, vasopressin secretion from the posterior pituitary is inhibited and results in the excretion of dilute urine, with subsequent return of the plasma osmolality to normal values. When plasma osmolality is increased, vasopressin release and thirst are stimulated and the combination of decreased urinary water loss and increased water intake results in decreased plasma osmolality. The influence of sodium/water balance on extracellular osmolality and the responses that aim to return osmolality toward normal values are shown in Figure 3.6.
In order to preserve a stable internal environment, there is a minimum volume of water that must be excreted by the kidneys, termed the volume obligatoire. This obligatory renal water loss is directly related to the excretion of solutes. If 800 mOsm of solute has to be excreted per day to maintain the steady state, and the maximum urinary osmolality is 1200 mOsm/kg, a minimum of 670 ml/day of urine will be required to excrete the 800 mOsm solute load. In the clinical setting of critical illness, where the concentrating ability of the kidneys may be compromised, it can be recognised that the volume obligatoire must increase in order to excrete an excess solute load.
The normal kidney responds to water or sodium excess or deficit, via osmo- and volume receptors, acting through vasopressin and the RAAS to restore effective circulatory volume and ECF osmolality. The receptors which facilitate the release of vasopressin include those sensitive to changes in osmolality located in the anterior hypothalamus, as well as pressure receptors relaying information from cardiovascular afferents to the nucleus of the tractus solitarius. Maintenance of volume always overrides maintenance of osmolality if hypovolaemia and hypoosmolality coexist. Vasopressin secretion from the magnocellular neurones of the supraoptic and paraventricular nuclei acts primarily on the kidney to increase urine osmolality. It achieves this through two main effects:
In addition to the actions of vasopressin, the RAAS works in parallel to conserve sodium and water. Synthesised and stored in the juxtaglomerular cells of the kidney, renin is released in response to low blood pressure in afferent arterioles, decreased tubular chloride concentration reaching the macula densa, or excitation of sympathetic nerves innervating the juxtaglomerular cells. The primary role of renin is to cleave angiotensinogen to angiotensin I. This in turn is cleaved to angiotensin II by angiotensin-converting enzyme. The physiological effects of angiotensin II are wide-ranging, but important actions for the control of sodium/water balance include the stimulation of aldosterone release from the adrenal cortex, reduction of renal blood flow, increased tubular sodium reabsorption, and the stimulation of thirst. Aldosterone is a steroid hormone which stimulates sodium and water reabsorption from the distal tubule and collecting-duct segments of the nephron. Providing negative feedback to inhibit renin release, and in turn the RAAS as a whole, are high circulating levels of angiotensin II, increased intrarenal arterial pressure, elevated tubular solute load, and tubuloglomerular feedback.
In addition to established roles in the control of blood pressure and circulatory volume, diverse effects of RAAS activation are increasingly being recognised, including those on the gastrointestinal system, where components of the RAAS influence the uptake and secretion of fluid and electrolytes. There also exist compounds derived from the intestine which are known to act on the kidney, principally to cause natriuresis, such as guanylin and uroguanylin.
Another peptide, urodilatin, which is produced by the renal distal tubules in a paracrine fashion, also stimulates natriuresis and has been shown to play a greater role in the response to elevations in sodium concentrations than atrial natriuretic peptide (ANP), which has been shown to respond primarily to changes in blood volume.
Control of effective circulatory volume
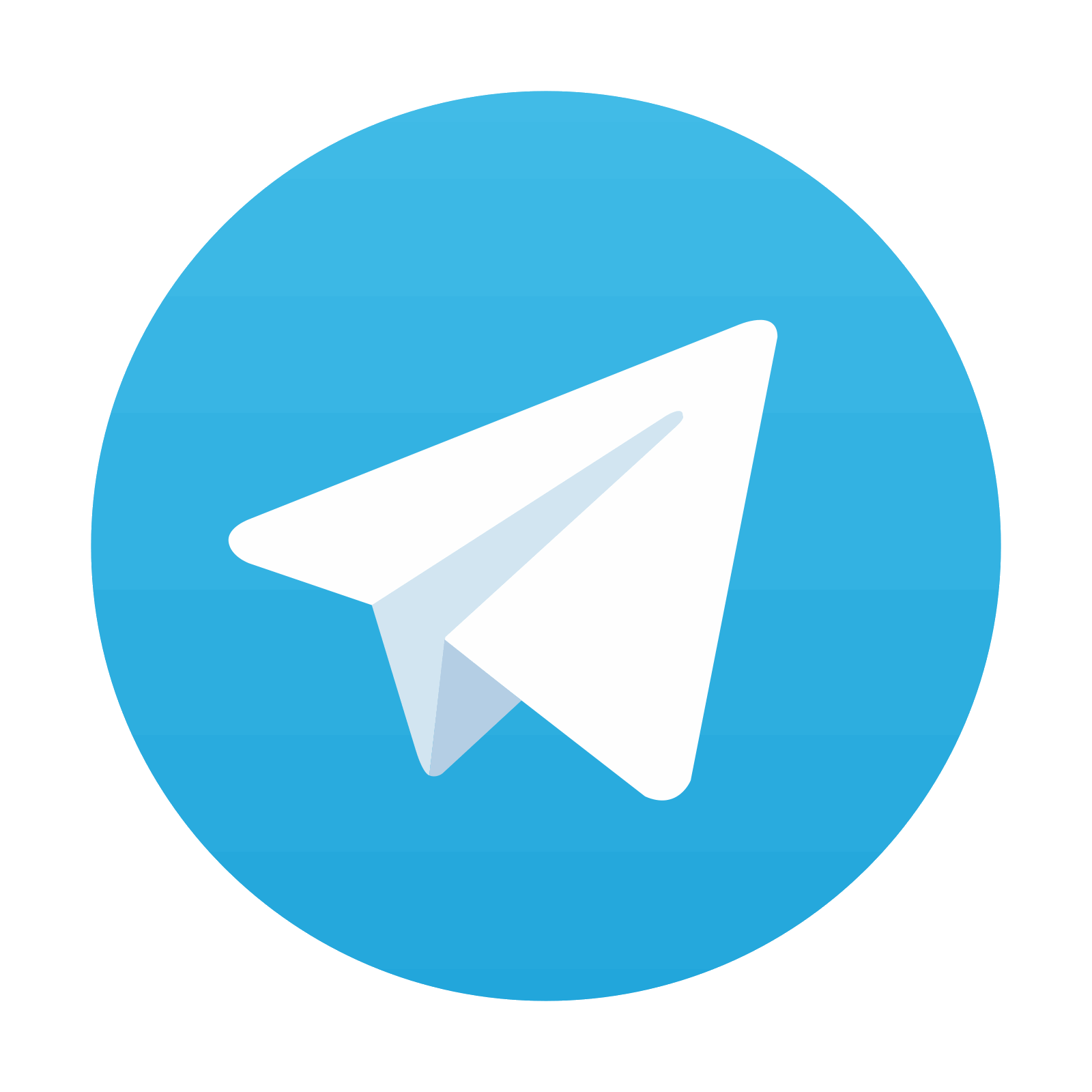
Stay updated, free articles. Join our Telegram channel
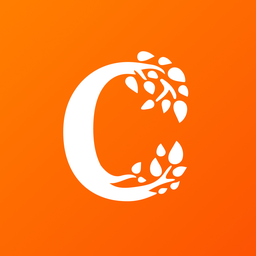
Full access? Get Clinical Tree
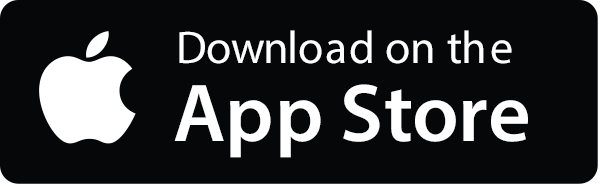
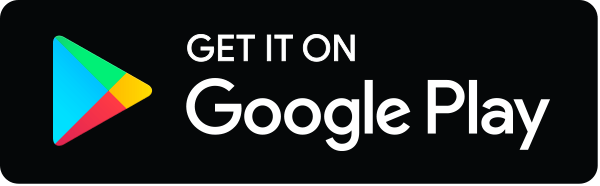