β-Thalassemia, originally named Cooley anemia, is an inherited blood disease. Various types of thalassemia are inherited anemias caused by mutations at the globin gene loci on chromosomes 16 and 11, affecting the production of α- or β-globin protein, respectively. The combination of early diagnosis, improvements in monitoring for organ complications, and advances in supportive care have enabled many patients who have severe thalassemia syndromes to live productive, active lives well into adulthood.
β-Thalassemia, originally named Cooley anemia, initially was described by Dr Cooley in 1925 in Detroit as an inherited blood disease. It is speculated that thalassemia was first recognized in the United States and not in its area of highest prevalence (the Mediterranean) because its presentation as a distinct clinical entity was masked by the fact that malaria, with its similar clinical picture of hemolysis, anemia, and splenomegaly, was ubiquitous in that region. Thus, patients who had this clinical triad were assumed to have malaria, not thalassemia. Now it is recognized that various types of thalassemia are inherited anemias caused by mutations at the globin gene loci on chromosomes 16 and 11, affecting the production of α- or β-globin protein, respectively.
The thalassemia syndromes are named according to the globin chain affected or the abnormal hemoglobin produced. Thus, β-globin gene mutations give rise to β-thalassemia and α-globin mutations cause α-thalassemia. In addition, the thalassemias are characterized by their clinical severity (phenotype). Thalassemia major (TM) refers to disease requiring more than eight red blood cell (RBC) transfusions per year and thalassemia intermedia (TI) to disease that requires no or infrequent transfusions. Thalassemia trait refers to carriers of mutations; such individuals have microcytosis and hypochromia but no or only mild anemia. Untreated TM uniformly is fatal in the first few years of life. In addition, TM and severe TI can lead to considerable morbidity affecting nearly all organ systems. The combination of early diagnosis, improvements in monitoring for organ complications, and advances in supportive care, however, have enabled many patients who have severe thalassemia syndromes to live productive, active lives well into adulthood.
Epidemiology
Similar to sickle cell disease and G6PD deficiency, the high prevalence of α- and β-thalassemia genotypes is believed a consequence of an evolutionary protection of heterozygotes against death from Plasmodium falciparum malaria. Before the twentieth century, thalassemia tracked with areas of malarial prevalence. β-Thalassemia arose in the Mediterranean, Middle East, South and Southeast Asia, and southern China. α-Thalassemia originated in Africa, the Middle East, China, India, and Southeast Asia. Immigration and emigration, however, have led to changing demographics, and patients who have thalassemia syndromes and heterozygote carriers now reside in all parts of the world. Thus, it is important for pediatricians, obstetrician, and hematologists to be aware of a possible diagnosis of thalassemia wherever they practice and for any patients they evaluate who have anemia. Consideration of the diagnosis allows proper diagnosis and management of individual patients and identification of carriers and ensures necessary testing and counseling to the population at risk for having children who have thalassemia.
Diagnosis
Understanding how thalassemia can be diagnosed requires a review of the structure of hemoglobin and the genetics of the thalassemia syndromes. Normal human hemoglobin is comprised of two α-like and two β-like globin chains. Adult hemoglobin consists of hemoglobin A (α 2 β 2 ) plus small amounts of hemoglobin A 2 (α 2 δ 2 ) and hemoglobin F (α 2 γ 2 ). Genetic mutations in one of the globin genes (α or β) result in decreased or absent production of that globin chain and a relative excess of the other. These mutations can result in no globin production (β° or α°) or decreased globin production (β + or α + ).
The α-globin gene is duplicated on chromosome 16; thus, each diploid cell carries four copies. The clinical syndromes of α-thalassemia reflect the number of inherited genes that are mutated. The α-thalassemia syndromes are silent carrier, α-thalassemia trait, hemoglobin H disease, and hydrops fetalis and reflect inheritance of 1, 2, 3, or 4 α-globin gene mutations, respectively. In contrast, a single β-globin gene resides on each chromosome 11. The four clinical syndromes of β-thalassemia, namely silent carrier, thalassemia trait, TI, and TM, correspond to the degree of expression of the two β-globin genes that encode β-globin and not the number of mutated genes.
α-Thalassemia has a wide spectrum of syndromes due to the possibility of one, two, three, or four allelic mutations. Mutation in one of the four alleles results in the silent carrier, with no clinical symptoms, normal complete blood cell count, and hemoglobin electrophoresis results past infancy. If two of the four α-globin alleles are mutated, affected individuals have α-thalassemia trait, with no clinical symptoms, but microcytosis and hypochromia and only mild anemia. The newborn screen often reports hemoglobin Bart, a fast-migrating hemoglobin that appears only in cord and neonatal blood when there is a deletion of one or more of the four α-globin alleles. Hemoglobin Bart is a γ 4 homotetramer that disappears rapidly in the neonatal period; its amount at birth corresponds to the number of affected alleles. Three α-gene mutations cause hemoglobin H disease with anemia characterized by microcytosis and hypochromia. Complete absence of α-globin chain production (all four alleles affected) leads to hydrops fetalis, which usually results in death in utero if intrauterine transfusions are not available.
β-Thalassemia has a similar spectrum of clinical phenotypes that reflect the underlying allelic mutations in the β-globin genes. If only a single β-globin gene is affected, then the resulting β-thalassemia silent carrier or trait results from partial (β + ) or absent (β°) gene expression, respectively. Similar to α-thalassemia trait, patients who have β-thalassemia trait typically have mild anemia, microcytosis, and hypochromia. When both β-globin genes are affected, then the resulting phenotype is more severe, depending on the degree of gene expression and relative imbalance of globin chains. For example, β + /β + genotypes typically are associated with an intermediate phenotype (TI), whereas the β°/β° genotype leads to the more severe TM.
Specific mutations in the α or β genes may lead to production of unique hemoglobins on electrophoresis, two of which have unusual features worth discussing in the context of thalassemia. Hemoglobin Constant Spring (Hb CS) is an α-globin gene variant caused by a mutation in the normal stop codon. The resulting elongated α-globin chain forms an unstable hemoglobin tetramer. Hb CS often occurs in conjunction with α-thalassemia so is associated with the more severe α-thalassemia phenotypes. Hemoglobin E (HbE) is caused by a nucleotide change in the β-globin gene, which leads to a single amino acid substitution (Glu26Lys) and diminished expression with a β + phenotype. HbE thus is an unusual “thalassemic hemoglobinopathy” that can lead to clinically severe phenotypes when paired with other forms of β-thalassemia.
Diagnosis
Understanding how thalassemia can be diagnosed requires a review of the structure of hemoglobin and the genetics of the thalassemia syndromes. Normal human hemoglobin is comprised of two α-like and two β-like globin chains. Adult hemoglobin consists of hemoglobin A (α 2 β 2 ) plus small amounts of hemoglobin A 2 (α 2 δ 2 ) and hemoglobin F (α 2 γ 2 ). Genetic mutations in one of the globin genes (α or β) result in decreased or absent production of that globin chain and a relative excess of the other. These mutations can result in no globin production (β° or α°) or decreased globin production (β + or α + ).
The α-globin gene is duplicated on chromosome 16; thus, each diploid cell carries four copies. The clinical syndromes of α-thalassemia reflect the number of inherited genes that are mutated. The α-thalassemia syndromes are silent carrier, α-thalassemia trait, hemoglobin H disease, and hydrops fetalis and reflect inheritance of 1, 2, 3, or 4 α-globin gene mutations, respectively. In contrast, a single β-globin gene resides on each chromosome 11. The four clinical syndromes of β-thalassemia, namely silent carrier, thalassemia trait, TI, and TM, correspond to the degree of expression of the two β-globin genes that encode β-globin and not the number of mutated genes.
α-Thalassemia has a wide spectrum of syndromes due to the possibility of one, two, three, or four allelic mutations. Mutation in one of the four alleles results in the silent carrier, with no clinical symptoms, normal complete blood cell count, and hemoglobin electrophoresis results past infancy. If two of the four α-globin alleles are mutated, affected individuals have α-thalassemia trait, with no clinical symptoms, but microcytosis and hypochromia and only mild anemia. The newborn screen often reports hemoglobin Bart, a fast-migrating hemoglobin that appears only in cord and neonatal blood when there is a deletion of one or more of the four α-globin alleles. Hemoglobin Bart is a γ 4 homotetramer that disappears rapidly in the neonatal period; its amount at birth corresponds to the number of affected alleles. Three α-gene mutations cause hemoglobin H disease with anemia characterized by microcytosis and hypochromia. Complete absence of α-globin chain production (all four alleles affected) leads to hydrops fetalis, which usually results in death in utero if intrauterine transfusions are not available.
β-Thalassemia has a similar spectrum of clinical phenotypes that reflect the underlying allelic mutations in the β-globin genes. If only a single β-globin gene is affected, then the resulting β-thalassemia silent carrier or trait results from partial (β + ) or absent (β°) gene expression, respectively. Similar to α-thalassemia trait, patients who have β-thalassemia trait typically have mild anemia, microcytosis, and hypochromia. When both β-globin genes are affected, then the resulting phenotype is more severe, depending on the degree of gene expression and relative imbalance of globin chains. For example, β + /β + genotypes typically are associated with an intermediate phenotype (TI), whereas the β°/β° genotype leads to the more severe TM.
Specific mutations in the α or β genes may lead to production of unique hemoglobins on electrophoresis, two of which have unusual features worth discussing in the context of thalassemia. Hemoglobin Constant Spring (Hb CS) is an α-globin gene variant caused by a mutation in the normal stop codon. The resulting elongated α-globin chain forms an unstable hemoglobin tetramer. Hb CS often occurs in conjunction with α-thalassemia so is associated with the more severe α-thalassemia phenotypes. Hemoglobin E (HbE) is caused by a nucleotide change in the β-globin gene, which leads to a single amino acid substitution (Glu26Lys) and diminished expression with a β + phenotype. HbE thus is an unusual “thalassemic hemoglobinopathy” that can lead to clinically severe phenotypes when paired with other forms of β-thalassemia.
Pathophysiology
The thalassemia syndromes were among the first genetic diseases to be understood at the molecular level. More than 200 β-globin and 30 α-globin mutations deletions have been identified; these mutations result in decreased or absent production of one globin chain (α or β) and a relative excess of the other. The resulting imbalance leads to unpaired globin chains, which precipitate and cause premature death (apoptosis) of the red cell precursors within the marrow, termed ineffective erythropoiesis. Of the damaged but viable RBCs that are released from the bone marrow, many are removed by the spleen or hemolyzed directly in the circulation due to the hemoglobin precipitants. Combined RBC destruction in the bone marrow, spleen, and periphery causes anemia and, ultimately, an escalating cycle of pathology resulting in the clinical syndrome of severe thalassemia.
Damaged erythrocytes enter the spleen and are trapped in this low pH and low oxygen environment; subsequent splenomegaly exacerbates the trapping of cells and worsens the anemia. Anemia and poor tissue oxygenation stimulate increased kidney erythropoietin production that further drives marrow erythropoiesis, resulting in increased ineffective marrow activity and the classic bony deformities associated with poorly managed TM and severe TI. Anemia in the severe thalassemia phenotypes necessitates multiple RBC transfusions and, over time, without proper chelation, results in transfusion-associated iron overload. In addition, ineffective erythropoiesis enhances gastrointestinal iron absorption and can result in iron overload, even in untransfused patients who have TI. It has long been recognized that the severity of ineffective erythropoiesis affects the degree of iron loading, but until the recent discovery of hepcidin and understanding, its role in iron metabolism the link was not understood.
Hepcidin, an antimicrobial hormone, is recognized as playing a major role in iron deficiency and overload. Hepcidin initially was discovered due to its role in the etiology of anemia of chronic inflammation or chronic disease. Elevated levels, associated with increased inflammatory markers, maintain low levels of circulating bioavailable iron in two important ways: (1) by preventing iron absorption and transport from the gut and (2) by preventing release and recycling of iron from macrophages and the reticuloendothelial system. Conversely, inadequate hepcidin allows increased gastrointestinal absorption of iron and ultimately may lead to excess iron sufficient to result in organ toxicity.
Iron not bound to transferrin, also referred to as nontransferrin-bound iron, damages the endocrine organs, liver, and heart. Nontransferrin-bound iron can result in myocyte damage leading to arrhythmias and congestive heart failure, the primary causes of death in patients who have thalassemia. Appropriate chelation therapy and close monitoring of cardiac siderosis can avoid this devastating complication (see the article by Kwiatkowski elsewhere in this issue for discussion of iron chelators).
Changing demographics and carrier screening
The clinical spectrum of thalassemia in the developed world has changed dramatically in the 3 decades since the introduction of deferoxamine chelation. In addition, new treatment options and prevention strategies to avoid complications of the disease and its compulsory treatments, coupled with immigration changes, have altered the demography of thalassemia in North America. Younger patients are now predominantly of Asian descent, whereas the aging population of patients who have thalassemia are of Mediterranean descent ( Fig. 1 ). According to the United States Census Bureau, the number of Asians increased significantly from 1980 to a total of 6.9 million in the census count in 1990. Coupled with other changes that have occurred over the past few decades, it is estimated that up to 100 million people of African, Hispanic, Southern and Eastern European, Middle Eastern, and Asian ethnic backgrounds reside in the United States. Similarly, it is estimated that approximately one sixth of the Canadian population is foreign born. This includes a considerable influx of Asians: in the 1990s more than 2 million people immigrated to Canada, approximately half from Asia. Many of the ethnic immigrants who relocated to North America are carriers of globin gene mutations, which have important implications for carrier screening. Recent reports reveal births of children who have severe α- or β-thalassemia in which appropriate screening and counseling was not offered to the parents.
Screening is inexpensive and simple but requires clinicians to be astutely attentive and aware of potential carriers. A complete blood cell count identifies microcytosis and hypochromia, which are present in nearly all thalassemia carriers at risk for having babies who have a severe thalassemia syndrome. In an adult, a mean corpuscular volume of less than 80 fL and mean corpuscular hemoglobin of less than 27 pg should alert a clinician to perform further screening, specifically hemoglobin electrophoresis and, in some cases, globin genotype testing. In addition, β-thalassemia carriers have elevated hemoglobin A 2 on adult electrophoresis unless there is concomitant iron deficiency, which may falsely normalize the hemoglobin A2 (HbA 2 ) level. Once confirmed, genetic counseling should be offered to parents or potential parents.
A newborn hemoglobinopathy screen can be helpful in the recognition and diagnosis of potential thalassemia syndromes. Severe β-thalassemia with absent β production due to two β° mutations has only fetal hemoglobin (HbF) as there is no hemoglobin A (α 2 β 2 ) produced. This abnormal “HbF only” result should prompt a clinician to follow the baby’s hemoglobin and growth to determine at what age the baby will need to initiate transfusions to prevent the complications of severe anemia. The anticipated severity of the α-thalassemia syndromes not always is determined easily by the results of a newborn screen. These babies all have an elevated percentage of hemoglobin Bart (γ 4 ) at birth but the level does not always correspond directly to the number of affected genes. This should alert pediatricians, however, to counsel parents to receive personal testing to determine the number of abnormal α-globin genes in each parent. If each parent has only one mutated α-globin gene, the most clinically significant outcome results in the birth of a baby who has a two-gene α-thalassemia (α-thalassemia trait), which has no clinical consequences. Conversely, if each parent has a two-gene mutation on the same chromosome, termed a cis mutation, there exists a risk for the most severe form of α-thalassemia, four-gene deletion, leading to hydrops fetalis, which often results in fetal demise without in utero transfusion.
Clinical complications
Transfusion-associated Issues
Iron overload
The primary long-term complication of chronic RBC transfusions for thalassemia is iron loading and the resultant parenchymal organ toxicity. Cardiac iron-overload leading to cardiac failure or arrhythmias is the most common fatal complication seen in chronically transfused patients who have thalassemia. Adherence to chelation can prevent cardiac damage and death from cardiac injury. Patients who present with cardiac arrythmias or failure due to iron injury often can be rescued by continuous infusion deferoxamine although adherence to the regimen of subcutaneous deferoxamine delivered 24 hours per day, 7 days per week, is difficult.
In addition, the endocrine organs are exquisitely sensitive to the toxic effects of iron and this may result in hypogonadotropic hypogonadism, pituitary damage, diabetes, osteopenia, and osteoporosis. Hypogonadotrophic hypogonadism is common in young adults who have TM and is believed to contribute to low fertility in this population. Additionally, cardiac complications of iron overload may exacerbate pregnancy and delivery complications in women who have thalassemia. Case reports and small published series reveal, however, that successful pregnancy and delivery of healthy babies is possible in women who have TM. Spontaneous pregnancy without hormonal assistance is reported. Recent literature on hypogonadotrophic hypogonadism suggests that early intervention with hormonal therapy and aggressive iron chelation therapy to prevent permanent damage may help preserve innate fertility. The effect of chelation on preservation of gonadal function currently is being investigated. For example, deferasirox, which provides extended blood chelator levels, may have a protective effect against toxicity to the endocrinologic organs, but further research remains necessary.
Alloimmunization
Chronic transfusions may result in the development of anti-RBC antibodies, alloantibodies and autoantibodies, in a variety of diseases. Although several studies have investigated the rates of alloimmunization in patients who had sickle cell disease, the thalassemia population is less well studied. Small retrospective analyses have suggested alloimmunization rates of 2.7% to 37% in patients who had thalassemia. Rates of alloimmunization are suggested as higher for transfusions with donor/recipient ethnic disparity and in splenectomized patients. Because of the risk for alloimmunization and autoimmunization, it is recommended that extended RBC antigen phenotyping be performed before initiation of RBC transfusions, so that patients can be transfused safely in the event of anti-RBC autoantibody or alloantibody formation. If a patient then develops autoantibodies directed against ubiquitous RBC antigens or multiple alloantibodies, blood that is matched more fully can be transfused more safely. For sickle cell patients requiring chronic transfusions, Rh and Kell antigen matching is considered standard of care and performed by many, but not all, care centers. For patients who have thalassemia, this matching strategy is not performed routinely. One recent study suggests, however, that matching for Rh and Kell in this population can decrease the alloantibody rate by 53%. Unlike most patients who have sickle cell disease, patients who have thalassemia usually initiate chronic transfusions at 6 months to 2 years of age. Many clinicians believe that chronic transfusions early in life may allow development of tolerance to foreign RBC antigens and prevent development of alloimmunization. Further prospective studies in this area are required to determine the appropriate transfusion strategy in patients who have thalassemia.
Viral infection
The transmission of infections, in particular HIV, hepatitis B, and hepatitis C, remains a serious complication and a significant problem in some developing countries. For now the sole use of volunteer donors who have no financial incentive to donate and thus are likely to answer a detailed donor questionnaire honestly provides the greatest protection against transfusion transmission of infections. Additionally, serologic and nucleic acid testing, used in the developed world, augment the safety of blood products. When these safety measures are in place, the risk for transfusion-transmission of known infections is extraordinarily low.
The development of the hepatitis B vaccine, identification of the hepatitis C virus (HCV), and a serologic test to screen donors has greatly minimized the risk for transfusion-transmitted hepatitis B virus and HCV. The prevalence of HCV in patients who have thalassemia is disparate and depends on the screening procedures and donor pool. In the developing world, the prevalence is 20% to 64%, with recent data demonstrating continued exposure and infection to patients who have thalassemia receiving transfusions, including many pediatric patients. In the North American population studied in the National Heart, Lung, and Blood Institute–sponsored Thalassemia Clinical Research Network, the prevalence of exposure was 70% in patients over 25 years of age but only 5% in patients under 15 years of age.
Chronic active hepatitis can lead to fibrosis, cirrhosis, and hepatocellular carcinoma (HCC) if untreated. Treatment with interferon-α and ribavirin is the standard of care for patients who have chronic hepatitis C. Because ribavirin causes hemolysis and thus increases transfusion requirements and concomitant iron exposure, the package guidelines for ribavirin still recommend that it not be used to treat chronic hepatitis in patients who are chronically transfused. Small studies have demonstrated, however, that ribavirin can be given safely and effectively to patients who have thalassemia. Because hepatic cirrhosis, liver failure, and HCC all are potential consequences of chronic active hepatitis, the majority of clinicians who care for these patients recommend treatment with interferon and ribavirin.
HCV-infected patients who have thalassemia are living long enough to develop prolonged chronic active hepatitis and be at risk for developing HCC. A multicenter retrospective review by Borgna-Pignatti and colleagues reported 22 patients who had HCC from a cohort of approximately 5000 patients who had thalassemia followed in 52 Italian centers. These numbers likely underestimate, however, the true risk for cirrhosis and HCC to patients who have thalassemia and are infected with hepatitis C, because many succumbed to cardiac complications before living long enough to develop frank cirrhosis or HCC. Patients who do not have evidence of hepatitis C exposure should have annual screening for HCV. Patients who have chronic hepatitis and are at risk for HCC should undergo routine screening with serum α-fetoprotein and liver ultrasound because survival in patients who have HCC is inversely proportional to the size of the tumor.
Thrombosis and Hypercoagulable State
Data compiled from many series present compelling clinical evidence for increased risk for thrombosis in patients who have β-TI, β-TM, α-thalassemia syndromes, or hemoglobin E/β-thalassemia. The risk for thromboses is increased in patients who are nontransfused or infrequently transfused and in patients who are splenectomized. This is believed to result in part from increased proportion of defective, innate RBCs. Defective RBCs have disrupted membranes resulting in exposure of negatively charged lipids, including phosphatidylserine, on the external cell surface, which are believed thrombogenic. In the absence of the splenic removal of senescent cells, more of these defective cells are circulating, which increases the risk for thrombosis. In addition, markers studied show increased platelet activation, which also is believed to increase thrombotic risk. The difficulty in ascertaining absolute clinical risk and determining appropriate preventive strategies is that many patients reported in the literature do not have exact transfusion regimens known. More frequent transfusions result in an increased ratio of normal transfused RBCs to disrupted innate RBCs, thus decreasing the clinical risk for thrombosis based on current knowledge of risk factors.
Data in hemolytic states, including sickle cell disease and thalassemia, suggest depletion of nitric oxide resulting from chronic hemolysis and increased plasma levels of free hemoglobin. Nitric oxide is a smooth muscle relaxant and decreased levels are believed to cause increased peripheral vascular resistance and ultimately pulmonary hypertension. Nitric oxide scavenging by free hemoglobin is implicated in the pulmonary arterial disease of sickle cell anemia and data suggest that this is a possible factor in thalassemia. Studies aimed at increasing the levels of nitric oxide and decreasing pulmonary hypertension are ongoing and will be critical in determining clinical approaches to this problem in the aging thalassemia population.
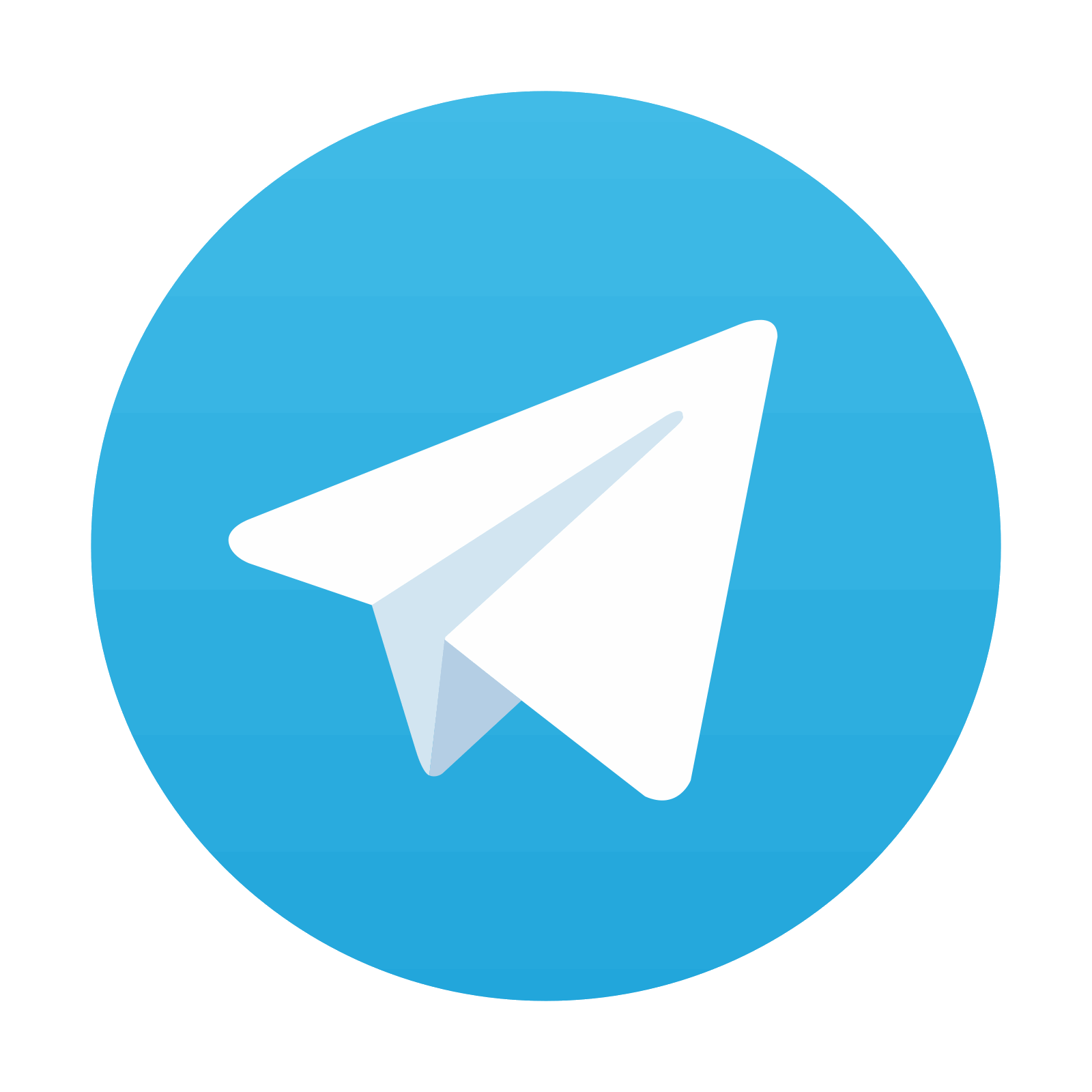
Stay updated, free articles. Join our Telegram channel
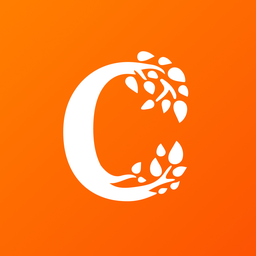
Full access? Get Clinical Tree
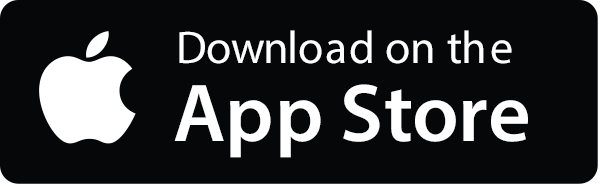
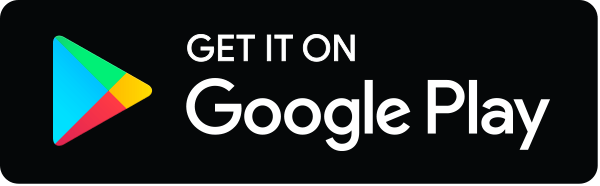