Total-Body and Hemibody Irradiation
Historically, total-body irradiation (TBI) has been used without stem cell support for palliation of radiation-sensitive disease such as chronic lymphocytic leukemia (CLL) or follicular lymphomas. TBI is mainly performed in the context of hematopoietic transplantation for its cytoxic and immunologic effects. Normal bone marrow tolerance for radiation is exceeded, and the patient’s hematopoietic system is reconstituted by the stem cell transplantation procedure. Donor cells may come from another human or from the patient’s pool of stem cells, referred to as allogeneic or autologous transplantation, respectively. In the case of donor stem cells from an identical twin, the term syngeneic transplantation is appropriate. Hemibody irradiation (HBI) has a different therapeutic goal, which is generally for palliation of diffuse metastatic disease. Stem cell support is not required.
TOTAL-BODY IRRADIATION
Historical Use of TBI without Stem Cell Rescue
Total-body irradiation has been used as a form of systemic therapy for various malignant diseases since the beginning of the 20th century.1 However, the usefulness of TBI without hematopoietic stem cell rescue is limited because the median lethal dose of whole-body radiation exposure given as a single fraction is approximately 4 Gy in humans. Given the radiosensitivity of chronic lymphocytic leukemia or low grade, advanced-stage non-Hodgkin lymphoma, TBI was an effective palliative modality using doses as low as 0.025 to 0.15 Gy several times a week, titrating total dose to clinical response.2,3 Because of myelosuppression—especially thrombocytopenia—the standard recommendation was to allow a 4- to 8-week treatment break after each cumulative 0.5 Gy of TBI.4,5 Johnson reported in the 1970s that one-third of patients with chronic lymphocytic leukemia attained complete remission with low-dose TBI alone, and slightly more did so when alkylating chemotherapy was added.5 These results were not supported by a Eastern Cooperative Oncology Group phase III trial, however,6 and the role for TBI without stem cell rescue has diminished further with advances in cytotoxic chemotherapy since the 1960s, as well as with the recent availability of anti-CD20 antibody therapies, including radioimmunoconjugates.
TBI in Stem Cell Transplantation
The conditioning regimen for hematopoietic stem cell transplantation has several functions. One is cytotoxicity: to contribute to the eradication of any residual cancer. Another important function of the conditioning regimen is immunosuppression so that the host does not reject the allogeneic donor stem cells. TBI in the broad range of 2 to 15 Gy in conjunction with chemotherapy serves these functions well.
Radiobiologic Effects on Normal Hematopoietic System
Successful hematopoietic stem cell engraftment requires (a) eradication of the recipient bone marrow, (b) immunosuppression to prevent rejection of donor marrow in the case of an allotransplant, and (c) relative sparing of the recipient’s bone marrow stromal cells. The reported D0 values of bone marrow stem cells usually range from 0.5 to 1.4 Gy, indicating intrinsic radiosensitivity.7,8 Although conventional wisdom assumes that recipient marrow cells must be removed to leave space for donor cells in stem cell microenvironmental conditions to favor the donor cells in a competitive repopulation, this concept has been challenged. In fact, mixed bone marrow chimerism resulting from a less cytotoxic nonmyeloablative transplantation may be acceptable or even desirable.
Immunosuppression in the setting of allogeneic bone marrow transplantation is necessary to avoid rejection of donor marrow, and TBI is a very efficient immunosuppressant. In animal work by Storb and colleagues, equivalent doses of fractionated TBI were significantly less effective than single-dose TBI to condition DLA-identical littermate dogs before bone marrow transplantation.9,10 Their conclusion was that there was significant repair of DNA damage by lymphoid cells during interfraction intervals. In a murine model, Salomon et al. looked at three TBI schemas from schedules that had been proposed for human TBI (8.5 Gy single-dose TBI, 2 Gy times 6 fractions of TBI, and 1.2 Gy times 12 fractions of hyperfractionated TBI).11 In terms of the immunosuppressive effects, the results favored single-dose TBI. A marked initial shoulder on the dose–survival curve has been reported for T-lymphocyte precursors12 and for a human lymphoblastoid cell line.13 There is a marked fractionation sensitivity of the immunosuppressive effect of TBI, leading one to conclude that fractionated TBI would lead to more graft rejections than the same dose delivered in a single fraction. Clinical data confirm these findings, in that fractionated TBI programs using total doses of 13 to 15 Gy are roughly equivalent to the efficacy of 10-Gy single-dose TBI.
If bone marrow stromal cells and their progenitors (fibroblast colony-forming cells) are damaged, delayed engraftment or even graft failure may follow.14 Progenitors of human bone marrow stromal cells have been found to have a D0 of 1.46 Gy.15 They are also sensitive to dose rate effects and fractionation; thus, fractionated TBI spares bone marrow stromal cells and their progenitors better than does single-dose TBI.16
Radiobiologic Effects on Leukemia
In the setting of stem cell transplantation procedures, TBI achieves significant leukemia cell killing and, in conjunction with chemotherapy and graft-versus-leukemia (GVL) effect, leads to eradication of malignant clones in a significant portion of cases. The use of the D0 value gives a rough indication of the radiosensitivity of various cell populations; most D0 values for both animal and human leukemias cell lines range from 0.8 to 1.5 Gy,17–18,19–20 although extreme values range from 0.3 to >5 Gy. Leukemic cell lines frequently show a minimal initial shoulder in radiation cell survival curves, leading to the hypothesis that fractionation (or reduced dose rate exposure) should have only a minor effect on cell survival. Split-dose radiation experiments lend further support to this hypothesis. Greater repair capacity is seen with more differentiated leukemias or lymphocytes (e.g., B- or T-cell phenotypes).
Graft-Versus-Leukemia/Tumor Effects
Allogeneic stem cell transplantation is largely an immunologic therapy. Allogeneic hematopoietic cells must be matched with the recipient for the majority of the major histocompatibility antigens to avoid rejection and minimize graft-versus-host disease, but minor human leukocyte antigen (HLA) differences facilitate a graft-versus-leukemia effect that enhances transplantation efficacy. Early studies demonstrated improved leukemia control with allogeneic bone marrow cells as compared to syngeneic (identical twin) donor cells. Further evidence for the graft-versus-leukemia effect derives from the efficacy of donor lymphocyte infusions after relapse of leukemia following allotransplant. In general with allogeneic transplantation, it is necessary to modulate the immune reconstitution during engraftment (e.g., using cyclosporine) to produce a graft-versus-leukemia effect while minimizing graft-versus-host disease.
Graft-Versus-Host Disease
Despite HLA matching, allogeneic stem cell transplantation is limited by graft-versus-host disease (GVHD). GVHD results from the activation and proliferation of mature donor T cells that recognize recipient alloantigens presented as peptide molecules by antigen-presenting cells (APCs). In the setting of allogeneic transplantation, despite HLA matching, a repertoire of peptides displayed on recipient cells can be recognized as minor histocompatibility antigens by donor T cells due to the polymorphisms in genes outside the HLA system.21 The activation of donor T cells after contact with specialized APCs leads to differentiation to effector cells that produce cytokines such as interferon-gamma and tumor necrosis factor, as well as mediate cytotoxicity against normal recipient organs. Acute GVHD includes clinical damage to skin, gastrointestinal tract, and liver, but other organs can also be involved. Later GVHD may present as a chronic form with more varied clinical symptoms similar to rheumatologic or connective tissue diseases.
Despite the prophylactic use of immunosuppressive agents such as cyclosporine, tacrolimus, methotrexate, prednisone, or mycophenolate mofetil, more than half of the recipients undergoing HLA-matched sibling hematopoietic transplants develop some degree of GVHD.22,23 Advanced forms of graft-versus-host disease require augmented immunosuppression, typically with high doses of corticosteroids, which place patients at higher risk for posttransplant infections.24 GVHD can be mitigated by T-cell depletion of the donor cells during the transplant procedure, but this then leads to an increased risk for infections, as well as to concerns that the desirable graft-versus-leukemia effects are diminished or lost. Nevertheless, there have been some clinical success with T cell–depleted allogeneic transplants in reducing GVHD, which typically relies on TBI-based myeloablative conditioning regimens.25,26
Recent laboratory work has focused on identifying T-cell subsets in the donor product that may be responsible for GVHD and differentiating them from T-cell populations that retain the important ability to mediate graft-versus-tumor effects and to reconstitute T-cell immunity to various viral and fungal pathogens such as cytomegalovirus, Epstein-Barr virus, Candida albicans, influenza, and varicella-zoster virus. So-called naive T cells appear to mediate GVHD and in humans have distinct cell surface antigens of CD45RA and CD62L.27,28 Selective depletion of naive T cells with specific retention of memory T-cell subsets in murine experimental models of allogeneic transplantation have successfully reduced GVHD while retaining graft-versus-leukemia effects.29–32,33 This observation has led to current investigations of selective T-cell depletion of CD45RA cells in the donor product along with fludarabine and TBI myeloablative conditioning. The hope is that T-cell populations including natural killer (NK) cells, NK/T cells, and central memory T cells will be retained to mediate important immunologic mechanisms of leukemia, tumor, and pathogen kill that improve the therapeutic ratio of transplantation.
TBI Dose-Limiting Toxicity—Pneumonitis and Other Late Effects
Early in the history of TBI, grade 4–5 solid-organ toxicities were found to be a major limitation, prompting a movement away from low-dose-rate, single-fraction TBI to fractionated regimens and non-TBI regimens. Studies in mice and humans show that the toxicities of TBI can be improved further by fractionating the radiation, as well as delivering by radiation at low dose rate. There is a high interfraction repair capacity of normal lung tissue. Thames and Hendry reported a low α/β value (3 to 6 Gy) for lung.34 This has been confirmed with both animal and human data.35–37 Sampath et al. estimated the α/β ratio for lung to be 2.8 Gy based on statistical modeling from 20 reports of clinical TBI in a total of 1,090 patients.38 The lung-sparing effect for fractionation has been shown to be important down to roughly 1 Gy.39 This dose roughly corresponds to the lowest fraction size in some hyperfractionated TBI schedules.40 The marked lung-sparing effect of fractionation or of a decrease in dose rate has been confirmed by the work of Penney showing a progressive sparing of the lung with increased fractionation for both early pneumonitis and late fibrosis.41 The rate of lung repair between fractions was reviewed by Travis, indicating the presence of two significantly different repair rates corresponding to a fast-repair half-time of 0.40 hour and a slow half-time of 4.01 hours.42 The slow-repair component needs to be kept in mind when designing TBI schedules that include two or three fractions per day.37
The reduction of the risk of pneumonitis by fractionation is supported by a randomized clinical trial comparing low-dose-rate, single-fraction TBI (9.2 or 10 Gy) with low-dose-rate, fractionated TBI (12 Gy in six fractions over 3 days) for patients with acute myelogenous leukemia in first remission, which showed a significant improvement in event-free survival with fractionation, mainly because of a reduction in early mortality. Interstitial pneumonitis in these patients was decreased from 26% to 15% with fractionation.43 Other studies confirmed that fractionated TBI regimens markedly reduce the incidence of idiopathic interstitial pneumonitis44,45–46 to less than 20% without increasing the rate of tumor recurrence. Within a range of conventional fraction sizes of 1.5 to 2 Gy given once or twice daily, no significant increase in the incidence of interstitial pneumonitis was noted up to total doses as high as 15 Gy.44,47,48 Nevertheless, total dose delivered to the lung is a key determinant of pneumonitis risk.49,50
Another radiobiologic approach to reduce the incidence of interstitial pneumonitis has been to lower the radiation dose rate—in essence a kind of continuous fractionation. Empirically this was found to be efficacious,51,52 but treatment times of 2 to 3 hours or more are impractical for most radiotherapy departments. Moreover, such long treatment times are poorly tolerated by patients. Fractionated TBI appears to be a better way of exploiting the potential advantages inherent in the different radiobiologic properties of tumor cells and the lung. Within the context of fractionated TBI schemes, instantaneous dose rates of 0.05 to 0.18 Gy/min have been generally used, often determined by the available output of linear accelerators at extended treatment distances. It is unclear whether higher instantaneous dose rates are detrimental, despite theoretical concerns in this regard. One small study that compared dose rates of 0.075 versus 0.15 Gy/min in context of a TBI prescription of 12 Gy in six fractions reported a pneumonitis risk of 13% versus 43%, respectively, although there were many confounding covariates.53 Other studies have not shown that dose rate is an important predictor of pneumonitis from fractionated TBI, but rather that total dose to the lung is the more critical determinant.38
Clinical Myeloablative Stem Cell Transplantation
Bone marrow or stem cell transplantation was conceived as a method of rescuing patients from the lethal effects of dose-intensive chemoradiotherapy. TBI has been a central part of allogeneic transplantation for leukemias ever since the pioneering work of Thomas and associates beginning in the late 1950s. A single dose of 9.2 Gy at a low dose rate of 0.07 Gy/min was required to obtain a consistent and sustained engraftment of allogeneic marrow in experimental animals. Consequently, a dose of 10 Gy at a dose rate of 0.07 to 0.10 Gy/min using cobalt teletherapy was used in humans.
The premise that TBI had to be given as a single fraction was challenged in the late 1970s when Peters et al. demonstrated the marked sensitivity of most normal tissues to altered fractionation and dose rate with minimal effects on bone marrow progenitors and leukemic cells.52,54 It was concluded that with the same total dose an improved therapeutic ratio would be expected from a reduction in the dose rate of single-fraction TBI or by TBI fractionation. Calculations of various fractionation schemes and dose rates have been published based on the linear quadratic model.55–57 O’Donoghue calculated that for very low dose rate TBI to be equivalent radiobiologically to the more common fractionated TBI schedules, an unreasonable radiation time of 20 to 24 hours would be necessary.57 With concepts of both radiobiology and practicality in mind, a large variety of fractionated TBI schedules have been used. After a generation of clinical investigation, no one regimen is clearly superior to another; so many confounding variables exist (including TBI technique, disease and patient heterogeneity, chemotherapy, supportive care, and immunosuppression) that it is impossible to clearly demonstrate the superiority of a particular regimen. At present, most myeloablative TBI programs use a twice- or three-times-daily fractionation scheme over 3 to 4 days to deliver a total dose of 12 to 15 Gy.
Chemotherapy Used with TBI
Chemotherapy agents used in conjunction with TBI include cyclophosphamide, etoposide, and cytosine arabinoside, with cyclophosphamide at 120 mg/kg over 2 days being the most common based on early work from E. Donnall Thomas and colleagues in Seattle. Programs adding other agents have been used primarily in high-risk transplant settings, but cyclophosphamide remains a backbone, given its effectiveness in immunosuppression and cytotoxicity. Reduced-intensity or nonmyeloablative transplant regimens using low-dose TBI that have been developed in recent years have often used fludarabine or pentostatin in place of cyclophosphamide.58,59 Chemotherapy and TBI are given sequentially rather than concurrently to avoid any potential increase in normal tissue toxicity. Whether chemotherapy should be given before or after TBI is unclear, and in the absence of clinical data showing which is best, logistic issues are the main consideration. Typically, TBI over 3 to 4 days is best delivered during the regular work week, when the full technical support staff is available. TBI may be better tolerated if given first when the patient is less fatigued and not sick from the effects of chemotherapy. The risk of nosocomial infections may be marginally lower when the patient travels to the radiotherapy department before becoming neutropenic later in the conditioning course. Alternatively, TBI at the end of the preparative regimen allows the stem cell transplant to proceed immediately thereafter; unlike chemotherapy, there is no washout period for elimination of the cytotoxic agent that would otherwise be harmful to the stem cells, thus saving a day or so of expected neutropenia while awaiting engraftment.
Non-TBI Conditioning Regimens
Although the evolution of TBI has led to significant reduction in toxicities, the initial concern about risk for fatal radiation pneumonitis lead to the development of non-TBI regimens. Santos at Johns Hopkins was the first to administer busulfan in place of radiotherapy in the busulfan–cyclophosphamide (BuCy) regimen.60 Subsequently, a reduction in the cyclophosphamide dose (BuCy2) reduced toxicity without an apparent compromise in efficacy, and this regimen was established as a standard alternative to TBI-based conditioning.61,62–63
There have been numerous comparisons of the BuCy conditioning regimen and TBI-based conditioning, including five prospective randomized studies, several meta-analyses, and registry review studies with large patient numbers.64–69,70,71,72–73,74–75 A French randomized clinical trial compared BuCy to cyclophosphamide-TBI before allogeneic bone marrow transplantation for adult acute myeloid leukemia (AML) in first remission.65 The results showed that cyclophosphamide-TBI was superior for disease-free survival, relapse, and transplant mortality. A similar randomized study by the Nordic Bone Marrow Transplant Group also showed superior survival and lower morbidity with TBI-based conditioning.64 In contrast, in chronic myeloid leukemia (CML) in chronic phase, the Seattle group demonstrated that BuCy was better tolerated and associated with a survival and relapse probability that was comparable to that of cyclophosphamide-TBI.67 In 2001 Socie et al. reviewed the pooled outcome of all patients in the aforementioned four randomized studies with a mean follow-up of 7 years.71 The probability for cure was statistically similar for both CML and AML, although a nonsignificant advantage for TBI in patients with AML was suggested. Long-term complications occurred equally following both types of conditioning, except that a higher risk of cataracts with TBI and irreversible alopecia with busulfan were noted. Registry reviews and meta-analyses typically show similar results for either regimen, with a possible advantage for TBI-based conditioning in AML and a higher risk of veno-occlusive disease of the liver in association with busulfan use.63,72–73,74–75
Studies on either side of the question have held very few variables constant, making the data almost impossible to interpret. Advances in the safe delivery of busulfan with pharmacokinetic monitoring and an intravenous formulation have further reduced the differences noted between conditioning regimens. The choice of conditioning regimen prior to transplantation depends upon a variety of factors that include the type of transplantation (allogeneic or autologous), the disease type, patient treatment history and condition, and the status of disease.
Toxicity Concerns Related to Choice of Conditioning Regimens
As TBI techniques have evolved, the risk of pneumonitis has become similar between TBI and non-TBI regimens. However, radiation factors are critical to keeping this risk of pneumonitis and other end-organ damage to a minimum. Not only attention to total dose, fractionation, and dose rate is important, but also dose homogeneity, organ dose reduction, and prior exposures, particularly from other cytotoxic therapies, must be considered. Prior thoracic radiotherapy is a risk factor for fatal pneumonitis (32% risk after TBI in patients with prior chest radiation doses >20 Gy in one study) and would be a reason to avoid myeloablative TBI.76 Prior chemotherapy exposures must also enter the equation in choosing a transplant conditioning regimen. One contemporary series of myeloablative transplants documented a distinctly high rate of pneumonitis in 33% of patients, in which the number of prior chemotherapy regimens was a significant risk factor for lung toxicity.77 (This study may have also highlighted the pitfalls of poor lung-dose homogeneity from the use of lateral TBI fields in adults using low-megavoltage photons in the 4- to 6-MV range, in spite of lung dose compensation.)
Historically, a significantly higher risk of hepatic veno-occlusive disease, hemorrhagic cystitis, and seizures has been associated with BuCy compared with TBI-based regimens.64,70,78–80 Advances in pharmacokinetic monitoring of busulfan blood levels and the advent of an intravenous formulation of the drug have been associated with reductions in hepatic toxicity and improved tolerability, even in older patient populations.81,82 A once-daily regimen of intravenous busulfan combined with the purine analogue fludarabine was associated with improved outcome compared to the BuCy2 regimen in a retrospective comparison.83
A relative advantage in using TBI for stem cell transplantation is that the dose delivery throughout the body is highly controllable. In contrast to chemotherapy, dose distribution is independent of such factors as blood supply, and there are no concerns about agent activation, metabolism, excretion, or dose modifications based on liver or kidney function. TBI may also reach chemotherapy sanctuary sites, which is of particular concern, for example, in patients at risk for or with central nervous system (CNS) involvement.
Historically, radiation oncologists have aimed to deliver a relatively homogeneous dose of TBI throughout the whole body, given the concern that leukemias are systemically distributed. Whether this is always an important goal is unclear, because microscopic disease burden during remission may not be uniform throughout the body. Some TBI programs use photon energies greater than 20 MV, which may theoretically deliver higher marrow doses due to increased pair production and higher bone absorption.84 Many correct for skin-sparing effects of megavoltage irradiation, for instance, with use of beam spoilers, although this is probably not necessary for low-energy photons in the absence of leukemia cutis or a trophism for skin involvement such as in monocytic leukemias. Where there is concern for a higher burden of disease, boost radiation fields may be added to TBI. Augmented doses of radiation may be delivered to the head in the setting of CNS relapse or prophylaxis or to the testes in males with ALL, as examples.
Clinical Data Regarding TBI Dose and Fractionation Schedule
A randomized study from Seattle in the setting of AML compared single-dose TBI (10 Gy) to a fractionated schedule (2 Gy times six fractions). The last update of this trial showed significant superiority of the fractionated scheme in terms of event-free survival.85 Investigators from France86 and Italy87 reported that dose rate did not influence the relapse rate. Another Seattle randomized trial of AML in first remission compared fractionated TBI doses of 12 Gy with 15.75 Gy, showing a decreased relapse rate from 35% to 12% but at the expense of a significant increase in therapy-related mortality, resulting in no survival advantage to a higher radiation dose.47 In short, in the setting of AML, (a) fractionated TBI appears superior to 10-Gy, single-dose TBI in terms of leukemia-free survival, and (b) dose rate has little impact on leukemia-free survival.
In contrast, a significant dose rate effect has been found for CML in chronic phase treated with allotransplant. A higher dose rate correlated with a decreased relapse rate.87,88 In addition, a multi-institutional, nonrandomized French study of 180 patients with CML showed that TBI fractionation was associated with an increase in relapse rate.89 In a French trial comparing busulfan/cyclophosphamide and cyclophosphamide/TBI, the actuarial risk of relapse was 11.1% after single-dose TBI (10 Gy) and 31% after fractionated TBI.69 The same trend is seen for patients receiving T cell–depleted marrow. In summary, a decrease in dose rate for single-fraction schemes or TBI fractionation in CML in chronic phase may lead to reduced leukemic cell killing.16
For ALL there is a paucity of clinical data regarding the optimal dose fractionation schedule for TBI. In a series from the City of Hope Medical Center, there was no significant difference in relapse rate between single-dose (10 Gy) and hyperfractionated TBI (1.2 Gy times 11 fractions over 4 days).90 A multicenter French study, however, showed a high likelihood of relapse for patients who received fractionated TBI with GVHD prophylaxis mainly via T-cell depletion.91 Together with elimination of GVHD, T-cell depletion also results in the loss of the GVL effect, which may unmask the known differences in antileukemic efficacy of TBI schedules that otherwise might be obscured by the combined efficacy of the conventional chemotherapy–irradiation–GVL association.92 In fact, there is evidence of both a dose-rate effect (less relapse with dose rates >14 cGy per minute) and fractionation effect (more relapse with fractionation), suggesting a repair capacity of some leukemic cells.16 A retrospective study from the Center for International Blood and Marrow Transplant Research and the City of Hope Medical Center reported improvement in outcome of patients with ALL in second remission when the dose of TBI exceeded 13 Gy.93
Nonmyeloablative or Reduced-Intensity Stem Cell Transplantation
In the last decade, growing recognition of the immunotherapeutic potential of allografts led to a reconsideration of the need for the high-dose myeloablative conditioning regimens traditionally administered prior to transplantation. Pioneering work of Storb and others in canine models established that highly immunosuppressive but nonmyeloablative regimens could establish stable mixed hematopoietic chimerism in major histocompatibility complex–matched littermates using one-sixth of the usual ablative dose of TBI in combination with postgrafting immunosuppressive drugs.9,94 Subsequently, numerous clinical trials have established that a spectrum of subablative conditioning regimens of varying intensity may allow engraftment of donor cells with reduction in regimen-related toxicity, permitting transplantation of patients traditionally excluded from allografting because of age or medical condition.59,95–99 In essence, allogeneic reduced-intensity transplantation is a form of immunotherapy. The primary purpose of the nonmyeloablative preparatory regimen is to suppress the patient’s immune system sufficiently to allow the engraftment of donor cells with minimum host toxicity. The graft-versus-tumor effect leads to eradication of tumor cells.
Common to these regimens is sufficient immunosuppression to overcome host resistance to engraftment using either antimetabolites such as fludarabine, TBI, or both in combination with other agents. Other factors, such as patient age, HLA disparity with the donor, tumor burden, and prior therapy, may also affect the degree of engraftment. A series of clinical studies by the Seattle transplant group, for example, demonstrated that a single 2-Gy fraction of TBI in combination with postgrafting cyclosporine and mycophenolate is sufficient to achieve a high rate of donor engraftment in patients with a prior history of autologous stem cell transplantation, but additional immunosuppression in the form of fludarabine is necessary to ensure engraftment in less heavily pretreated patients or patients receiving unrelated donor grafts.
Reduced-intensity transplants now account for approximately 25% of all allotransplants being performed. Efficacy is difficult to evaluate in the absence of randomized trials, but reduced-intensity conditioning regimens have allowed for an expanded use of transplants in high-risk populations. As an example, elderly patients with acute leukemias in first remission have long-term survival rates of greater than 40% with nonmyeloablative transplants, which is a remarkable achievement compared to historical experience, in which very few patients would have been expected to survive.100 Toxicity is significantly lower than with traditional myeloablative transplants. The Seattle group reported a 1-year non–relapse-related mortality of allotransplants of 30% for ablative regimens compared to 16% for reduced-intensity conditioning regimens (p = .04).101 Certainly based on first principles, the toxicity of a single dose of 2 Gy of TBI should be minimal. Most transplant centers delivering this dose of TBI would dispense with the complexities of lung blocks, as well as with compensating filters to optimize dose homogeneity. Intensity-modulated radiotherapy is being investigated for total-body irradiation.102–111 In one form using helical tomotherapy, whole-body marrow and lymphoid compartments are targeted while other organs are relatively spared from radiation exposures. The hypothesis is that the whole body does not need to be uniformly treated. Moreover, in the application of reduced-intensity transplantation, the important targets for radiotherapy may be the marrow to kill residual malignant cells felt to be in higher density in that hematopoietic compartment and the lymphoid tissues to effect immunosuppression. Of course, malignant cells may be in circulation or elsewhere in the body; statistical modeling of circulating cells suggests that a reasonable radiation dose may still be expected to be delivered to the blood pool with tomotherapy.112 However, one warning for this approach comes from an older clinical trial of single-fraction TBI with varying lung blocking, which showed that excessive lung shielding may reduce leukemia control.113 Nevertheless, there are early data employing total marrow and lymphoid irradiation (TMLI). A protocol at the City of Hope Medical Center combined fludarabine, melphalan, and TMLI to 12 Gy in eight fractions over 4 days. Dose to the brain, lungs, heart, intestines, kidneys, and bladder are specifically reduced. In a cohort of 33 patients with a variety of high-risk hematologic malignancies, this form of tomotherapy was found to be feasible and reduced median lung doses to 5.7 Gy compared to the expected 8 to 9 Gy with conventional techniques. Acute mucositis was still a significant problem, and the 1-year event-free survival rate was 72%.114 Further trials need to sort out whether this is a better method of using radiotherapy for hematopoietic transplantation.115
Total-Body Irradiation Technique and Dosimetry
Basic Requirements
Given the concern that leukemias are systemically distributed, TBI techniques have been designed historically to deliver a relatively homogeneous dose throughout the whole body. Because the large variations in body geometry and tissue density, the requirement on whole-body dose uniformity has been stated typically as within a specified window, for example, ±10%, of a prescribed dose, except in regions where additional local dose tailoring is planned for the protection of critical organs, such as the lungs, and/or for intensifying target cell kill, such as in bone marrow and lymphocytes, as dictated by clinical presentations. To ensure that a planned dose distribution is accurately delivered to TBI patients, all TBI techniques must undergo a rigorous and comprehensive dosimetric characterization. In the context of general radiotherapy, the International Commission of Radiation Units and Measurements has recommended an overall accuracy in dose delivery of ±5% based on analysis of dose–response data and evaluation of errors in dose delivery. For TBI and HBI, there is evidence that a 5% change in lung dose could result in 20% change in the incidence of radiation pneumonitis, a complication that is usually fatal for whole-lung irradiation.50,116 Therefore, the basic dosimetry of TBI and HBI techniques should be performed as precise as readily achievable.117 Accurate dosimetry coupled with an effective quality assurance program will ensure not only safe delivery of TBI treatments, but also accurate dosimetry data for meaningful dose–response analysis. Because some clinical procedures, such as bone marrow transplantation, require TBI at a specified time within a comprehensive drug and radiation treatment protocol, once a patient begins a course of TBI the timing of successive fractions becomes critical to the outcome of the procedure. It is imperative to plan a backup TBI system either within the same institution or in a nearby radiation therapy department when establishing a TBI program. When the primary system is down, a fully commissioned backup TBI system can complete the remaining treatment.
Total-Body Irradiation Techniques
Many techniques have been described in the literature for effective irradiation of the whole body, and, indeed, improvements in both the irradiation technique and physical dosimetry are still being reported.118–124 Much of the early clinical experience with TBI and HBI procedures was obtained at centers with facilities designed specifically for large-field irradiation.125 Although a few dedicated systems still exist, current TBI procedures are largely performed with techniques established on linear accelerators that are used for conventional radiotherapy. Common to these TBI techniques is the use of radiation fields that are larger than the maximum field size (~40 by 40 cm2) available at standard source-to-surface distance (SSD) treatment distance (~100 cm) by treating TBI patients at extended SSD of 200 to 600 cm. For treatment rooms larger enough to accommodate SSD of 5 m or greater, a single square field at maximum collimator opening will be sufficient to completely encompass patients of typical height placed along the diagonal of the field (Fig. 15.1 A–E). At shorter SSDs, multiple abutting fields are necessary (Fig. 15.1 F–H), and the irradiation of the whole body can be achieved by translating the radiation field126 or the patient127 or by sweeping the radiation field over a stationary patient.128 For these irradiation techniques, patients are typically treated with two parallel-opposed fields. When a single radiation source is used, this can be accomplished by rotating the patient 180 degrees along the patient’s longitudinal axis between the two irradiations. In a dedicated system with two radiation sources mounted opposite to each other, the treatment can be accomplished by irradiating the two fields simultaneously without changing the patient’s position. Various patient positions, ranging from sitting or standing upright to lying horizontally in supine or lateral decubitus positions,129,130 have been used in these techniques (Fig. 15.1). The technique using a single large field encompassing the entire patient at extended SSD is by far the simplest and the most prevalent TBI technique used today. The treatment is typically delivered with a horizontal field directed toward the primary shielding wall. It eliminates the dosimetry complications occurring in the junctions of multiple abutting fields. It also alleviates the concern that cells circulating through the body may potentially receive a reduced dose when abutting fields are delivered sequentially.
FIGURE 15.1. A–E: Some of the current large-field total body irradiation (TBI) techniques in which patient and beams are stationary: A: Two vertical beams. B: One vertical beam. C: One horizontal beam, patient in supine position. D: One horizontal beam, patient standing or sitting. E: One horizontal beam; patient in lateral decubitus position. F–H: Some of the small-field TBI techniques in which patient or beam moves. F: Source scans horizontally. G: Patient moves horizontally. G: Sweeping beam.
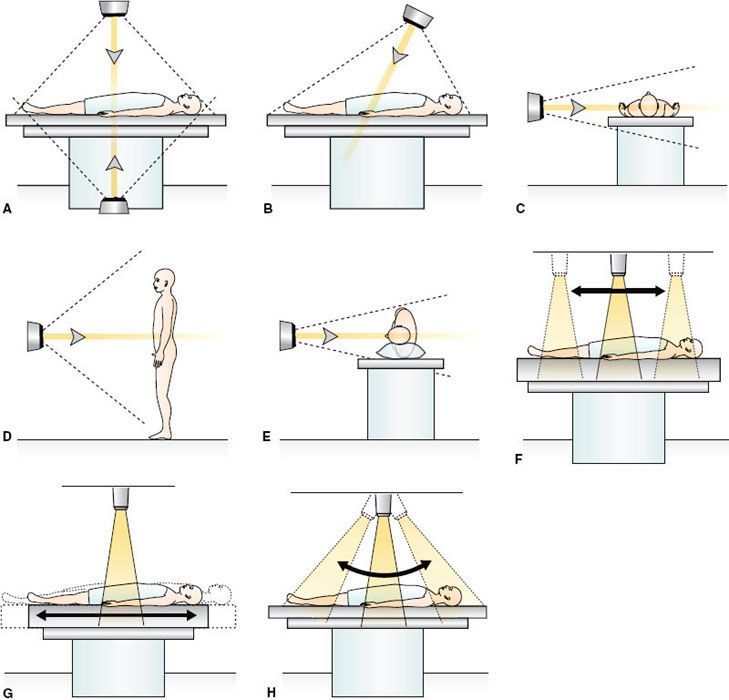
Recently, with the introduction of intensity-modulated radiation therapy (IMRT) and advanced IMRT delivery systems such as volumetric modulated arc therapy (VMAT) on conventional linear accelerators and spiral tomotherapy on dedicated treatment units, the possibility of delivering TBI-type of treatments at SSDs of conventional radiotherapy has been explored by several research groups.122–124 In addition to obviating the need of using extended SSD, these new techniques open the possibility of designing and delivering customized dose distributions throughout the whole body. Their ability to seamlessly deliver integrated boost dose to total marrow and/or lymphatic system while keeping doses to uninvolved critical organs low has the potential to further improve the therapeutic ratio of TBI treatment. Only limited clinical experience has been reported.131 More carefully designed and controlled clinical testing of these new techniques is needed to fully establish its clinical utility and efficacy.
Dosimetric Characterization of Total-Body Irradiation Techniques
Once an irradiation technique is chosen, a careful characterization of the dosimetric properties of the technique should be performed by a qualified medical physicist. The dosimetric data needed to model the treatment planning system for accurate planning of TBI for individual patients should be carefully measured and validated. The technical issues and method of radiation dosimetry for TBI has been reviewed in several reports.125,132,133 In particular, the report of American Association of Physicists in Medicine (AAPM) Task Group 29 (TG-29) on the physical aspects of total and half-body photon irradiation provides a comprehensive discussion on dosimetry issues and techniques specific to large-field TBI.125 It is a good resource for medical physicists charged to commission a large-field TBI technique.
Since the publication of TG-29 report, the reference dosimetry protocol for external beams at standard treatment SSD, known as the TG-21 protocol, has been updated by a new calibration protocol (TG-51). For photon beams at standard SSD, the TG-51 protocol produces similar results as the TG-21 protocol. However, the TG-51 protocol cannot be applied directly for large fields at extended SSD as encountered in TBI. AAPM has established a working group on dosimetry calibration protocol for beams that are not compliant with TG-51. This working group is charged to develop standardized procedures for calibration of noncompliant beams, among other assignments. In the meantime, the calibration of a TBI beam can be established with direct traceability to TG-51 by using an approach similar to that proposed by Curran et al.134 In this approach, the photo source of the TBI beam is calibrated first under the TG-51 reference condition at standard SSD. The dose per unit beam-on time at a reference point of the TBI beam under TBI treatment condition is then related to the dose per unit beam-on time of the same photon source at the TG-51 reference point by a correction factor that accounts for the TBI setup geometry and scattering conditions. This correction factor, as well as the relative dose factors that characterize the spatial distribution of the TBI beam, such as the percentage depth dose (PDD) or tissue maximum ratio (TMR) along the central axis, can be measured directly under the TBI treatment conditions using a phantom with size similar to that of a typical patient. In-phantom off-axis beam profiles at various depths, especially along the diagonal near the corners of the field, should also be measured at TBI treatment distance to evaluate the dose variation across the radiation beam. Independent verification of the TBI calibration should be performed after the initial commissioning. The modeling of treatment planning system should also be verified on an anthropomorphic phantom.135–138 A thermoluminescent dosimeter calibrated on an independent linear accelerator can be used to verify TBI calibration and doses at other points of interest. Ion chamber and film may be used to assess dose distributions.139
Skin Surface Dose
Although skin sparing is often a desirable feature of megavoltage irradiation in conventional radiotherapy, for TBI it may be desirable to have skin surface receive close to full prescription dose, as leukemia may circulate through or infiltrate the skin. When needed, the skin dose can be increased by using either bolus placed on the skin or a beam spoiler positioned between the source and the patient.140 In the latter technique, a large plastic screen (e.g., 2-cm-thick acrylic plastic sheet or acrylic resin) covering the whole body is placed approximately 10 cm from the patient. As the photons of TBI beam pass through the beam spoiler, scattered electrons are produced, which deposit most of their energy at shallow depths near the skin surface. The use of a beam spoiler alters the depth–dose characteristics of the TBI beam in the buildup region. The magnitude of this modifying effect depends on the photon energy of the TBI beam, the composition and thickness of the scatter screen, and the distance between the screen and patient. It should be carefully evaluated as part of the commissioning task for the TBI technique. The dosimetric effect of the beam spoiler can be treated separately or included in the TBI beam calibration. When the beam spoiler is included in the calibration, choice of the calibration depth becomes an important consideration. Calibration measurements performed at a depth of 5 cm or greater decrease the influence of beam spoiler–generated electrons significantly.
Dose Rate
As discussed in preceding sections, the rate of TBI dose delivery could have an impact on the biologic effects of TBI, depending on the disease.141 Many clinical protocols require low-dose-rate treatment at the rate of 0.05 to 0.10 Gy/min.125 Modern linear accelerators offer a wide range of dose rates, for example, from 1 to 6 Gy/min in steps of 1 Gy/min, to the depth of maximum buildup at standard SSD. At extended SSD, the nominal dose rate will be smaller due to inverse-square falloff of photon fluence with SSD. The dose rate at the TBI treatment distance is dependent on the combination of SSD and the nominal dose rate programmed at the linear accelerator (LINAC) console. If a given combination of SSD and nominal LINAC dose rate does not produce a desired dose rate at TBI patient, a custom-made attenuator can be placed in the beam path to help achieve a desired dose rate. TBI calibration and dosimetry characterization should be performed with the desired treatment dose rate to ensure accurate dose delivery.125
Patient Positioning
Because treatment times may last up to 30 or 40 minutes for each fraction of a fractionated TBI protocol (even longer for single-fraction protocols), patients must be placed in a comfortable and reproducible position. When a pair of parallel-opposed fields is used, irradiation along the anterior-posterior/posterior-anterior (AP/PA) direction is generally preferred, as the body thickness in the AP/PA direction is usually smaller than in the lateral direction, which would result in better dose uniformity along the beam path for a given photon energy. As depicted in Figure 15.1, this may be accomplished with patient lying in the supine/prone position under the vertical beam arrangement or with the patient lying on the side in decubitus position when a horizontally directed beam is used. Irradiation with patient lying in supine position using a horizontally directed beam is feasible for patients with small lateral separations, such as pediatric patients, or when a high-energy photon beam is employed. Because of clinical problems associated with patient fatigue and orthostatic hypotension, special patient stands are used in some institutions to facilitate upright patient positioning.
Treatment Planning
The calculation of radiation beam-on time for a prescribed dose and treatment planning for TBI are often performed with a specialized in-house program or by manual computation. This is because most commercial treatment planning systems designed and commissioned using standard data sets for conventional radiotherapy do not automatically apply to TBI configurations. Some new versions of commercial treatment planning systems can be adapted for isodose planning of TBI at extended SSD by using depth doses, beam profiles, and other parameters measured directly under the TBI condition. For example, a special TBI beam model was successfully commissioned on the Theraplan Plus 3D system and used in routine TBI treatment planning at Yale. Others have commissioned and evaluated an extended SSD photon model on the Pinnacle3 planning system for TBI.142 Newer techniques for total marrow and/or lymphatic irradiation using tomotherapy or VMAT at standard treatment distance can take advantage of the beam models already commissioned in an existing treatment planning system, although the dosimetric accuracy must undergo a careful validation for irradiating large and complex target volumes demanded by TBI/total marrow irradiation (TMI).
In addition to TBI beam characteristics, an accurate description of patient geometry is needed for patient-specific treatment planning. The external body contour of a TBI patient in treatment position could be reconstructed from the measurements of body thickness at representative anatomic points judiciously distributed over the patient body. Recently, computed tomography (CT) scan of the whole body became feasible. It provides the best description of patient geometry and is required for the new IMRT-based TBI/TMI techniques. For conventional extended SSD TBI techniques using two parallel-opposed beams, dose variation over the patient body arises primarily from (a) photon attenuation along the beam path, (b) changing body contour across the patient, and (c) variations in tissue density.
The dose variation caused by photon attenuation along the beam path is dependent on both the beam energy and the body thickness. It decreases with decreasing body thickness and increasing photon energy. Because the body is typically thinner in the anterior–posterior direction, treating patients using the AP/PA technique with higher photon energy will improve dose uniformity along the beam path. Using lateral opposed beams will usually result in greater dose variation compared to AP/PA treatments, especially for adult patients. The dose variation caused by changing body contour may be reduced by using a missing-tissue compensator or tissue-equivalent bolus material placed directly on the patient. The ability to compute isodose distribution across the body is highly desirable for compensator design. A missing-tissue compensator for TBI is typically constructed to even out the variation of body thickness along the head-to-toe direction. Such a one-dimensional compensator can be constructed manually using multiple thin copper (or other material) plates. Because the compensator is usually mounted at the head of the linear accelerator, small variations in the placement of compensating plates will be magnified at the extended SSD distance. Care must be exercised in constructing these compensators. For example, when compensating plates are used for the head and neck region, mounting the compensator too far inferiorly could result in underdose to the shoulders. In addition, careful alignment of patient to the planned position becomes important to achieve the desired missing-tissue compensation.
Dose variation caused by tissue heterogeneity in the thoracic region requires special attention because the lungs are a critical dose-limiting structure in TBI. Without compensation for air density, particularly for AP/PA treatments, dose inhomogeneity can exceed the prescribed dose by 10% to 24%, depending on the energy of beams used.125 To reduce lung toxicity, correction for lung air density using lung blocks is commonly used to reduce the dose to whole lung. The use of lung blocks increases the complexity of the TBI procedure, and accurate repositioning of lung blocks can be a challenge for fractionated treatments. Several techniques have been reported to increase the repositioning accuracy of lung blocks.143–145 At Yale, individualized thin lung blocks (with ~85% photon transmission) are mounted close to the patient on an acrylic resin tray using a hook-and-loop fastener system that allows easy repositioning of lung blocks for each fraction (Fig. 15.2C, D). Verification of correct lung block positioning is carried out by using a customized online electronic portal imaging. For the lateral technique, the arm can be used to shield the lungs, thereby improving dose homogeneity. Care must be taken to cover the lung with the arm. For pediatric patients, the arm may not be large enough laterally to cover the entire lung.
Dose Description and Reporting
Because there is no standard treatment technique for TBI and HBI, significant differences in dose distributions can exist with different treatment methods. Two institutions can prescribe the same dose at some selected prescription point, but dose to other points could vary considerably if different treatment techniques are used. Without supplemental information on the dose distribution, it would be difficult to assess the clinical effectiveness of different TBI programs based on the reported prescription dose alone.
To facilitate treatment comparison among institutions, various methods for prescribing the dose for TBI treatments have been reported.146,147 One method uses a single-point prescription dose supplemented with the specified limits of highest and lowest dose levels acceptable for any point within the body. In addition, dose limits are also set for certain specific tissues such as the lungs. An example of such a TBI prescription is given in AAPM TG-29, which uses the midpoint at the level of the umbilicus as the prescription point. A prescription would be read as follows: “The dose to the midpoint at the level of the umbilicus is 14 Gy to be delivered in eight fractions with two fractions on each day separated by at least 6 hours. All points in the body should receive doses within the limits of −5% and +10% of prescription dose. The dose to lung should be no more than 85% of the prescription dose.” When reporting TBI experience, the actual value of the corresponding dose-prescription descriptors achieved by the treatment plan should be provided. When CT-based TBI treatment planning is available, a dose–volume histogram of the target volume and critical organs should be reported.
Quality Assurance
To improve the dosimetric accuracy and consistency, periodic check of TBI calibration and beam characteristics should be performed as a part of an ongoing quality assurance program. Each patient’s treatment plan, including the design of customized tissue compensation filter when used, should be checked by an independent physicist. In addition, in vivo dosimetry verification of the treatment plan should be performed on the first fraction for all TBI patients. Changes in patient body shape (e.g., due to weight loss between the time of simulation and treatment delivery) and in positioning can alter the dose distributions. Adjustment of radiation beam-on time and of tissue compensation filter may be needed for the subsequent treatments based on the in vivo verification (assuming the accuracy and confidence of in vivo measurement have already been established). Thermoluminescent dosimeters and diode detectors are typical choices for in vivo monitoring of doses delivered to patients.138,139 These detectors should be calibrated in the TBI beam under the treatment condition prior to commencing the patient treatment. A diode dosimetry system with multiple diode detectors is especially convenient for this type of measurements because they allow simultaneous measurement of doses at multiple anatomic sites in nearly real time. At Yale, in vivo dose verification is performed using such a system for each patient on the first treatment fraction. Adjustments made to the treatment plan are verified in the following treatment fraction when necessary.
Lung (and Other Organ) Dose Attenuation
Many transplant centers use lung blocks during TBI in order to correct for the dosimetric effects of lung density or to specifically reduce the dose to a majority of lung tissue, thereby reducing the risk for pneumonitis. This is particularly important in patients who have baseline lung dysfunction.148 Lung shielding will clearly reduce the risk of pneumonitis, all other factors being equal.38 Overcompensation, however, risks an increase in leukemia recurrence.113 Specifically, a study from the Institut Gustave-Roussy delivering 10 Gy as a single fraction of TBI over 4 hours, showing a higher incidence of relapse in patients whose lung dose is limited by lung blocks to 6 instead of 8 Gy.113 The technique at Yale uses 1/8-inch lead filters that attenuate the dose by 10% to 15%, in essence a slight overcorrection for the dosimetric effects of pulmonary air density149 (see Figure 15.2). The Memorial Sloan Kettering Cancer Center group uses 1–half-value layer (HVL) shielding,45 with the use of electron boosts of the chest wall under the lung blocks. There are considerable dosimetric problems with this technique: treatments are planned in supine position but delivered standing; there are overlap issues of photon and electron fields; there is surface contour variability, especially from breast tissue in women; and there is still delivery of unwanted radiation dose to some limited volume of lungs. The Institut Gustave-Roussy reported no clinical benefit to electron boosts to chest wall under such lung blocks.150 The Johns Hopkins group reported using thick (7 HVL) blocks for just one fraction of their TBI course over several days.151 The Seattle group uses 1- or 2-HVL blocks for half of the TBI fractions without a chest wall boost. Other transplant groups, such as at the one at the University of Minnesota, have reported using partial transmission blocks to the liver and kidneys to reduce the risk of hepatic veno-occlusive disease or nephropathy.152,153 When TBI is used for nonneoplastic diseases (e.g., aplastic anemia), for which the main objective is immunosuppression, one may also consider shielding radiosensitive structures such as the gonads or eyes (i.e., the lens).154
FIGURE 15.2. A: Patient in decubitus position for total body irradiation (TBI), anterior beam. B: Thin lung blocks placed close to thorax using a Velcro–plexiglass system to reposition blocks with each fraction. C, D: Thin lung blocks (1/8-inch lead) used at Yale to attenuate dose under block by 10% to 15%, primarily to compensate for air density inhomogeneity in TBI dosimetry. C: A megavoltage simulation film taken in decubitus position. D: Treatment portal imaging.
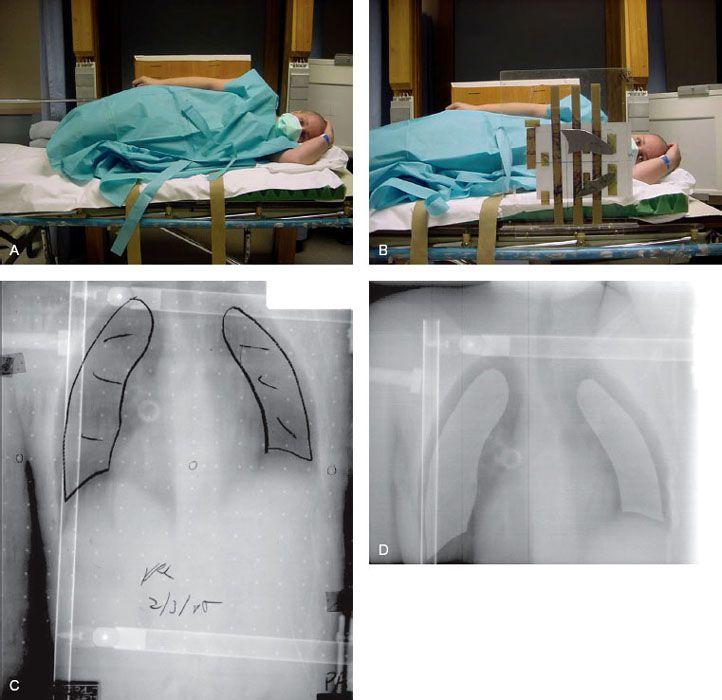
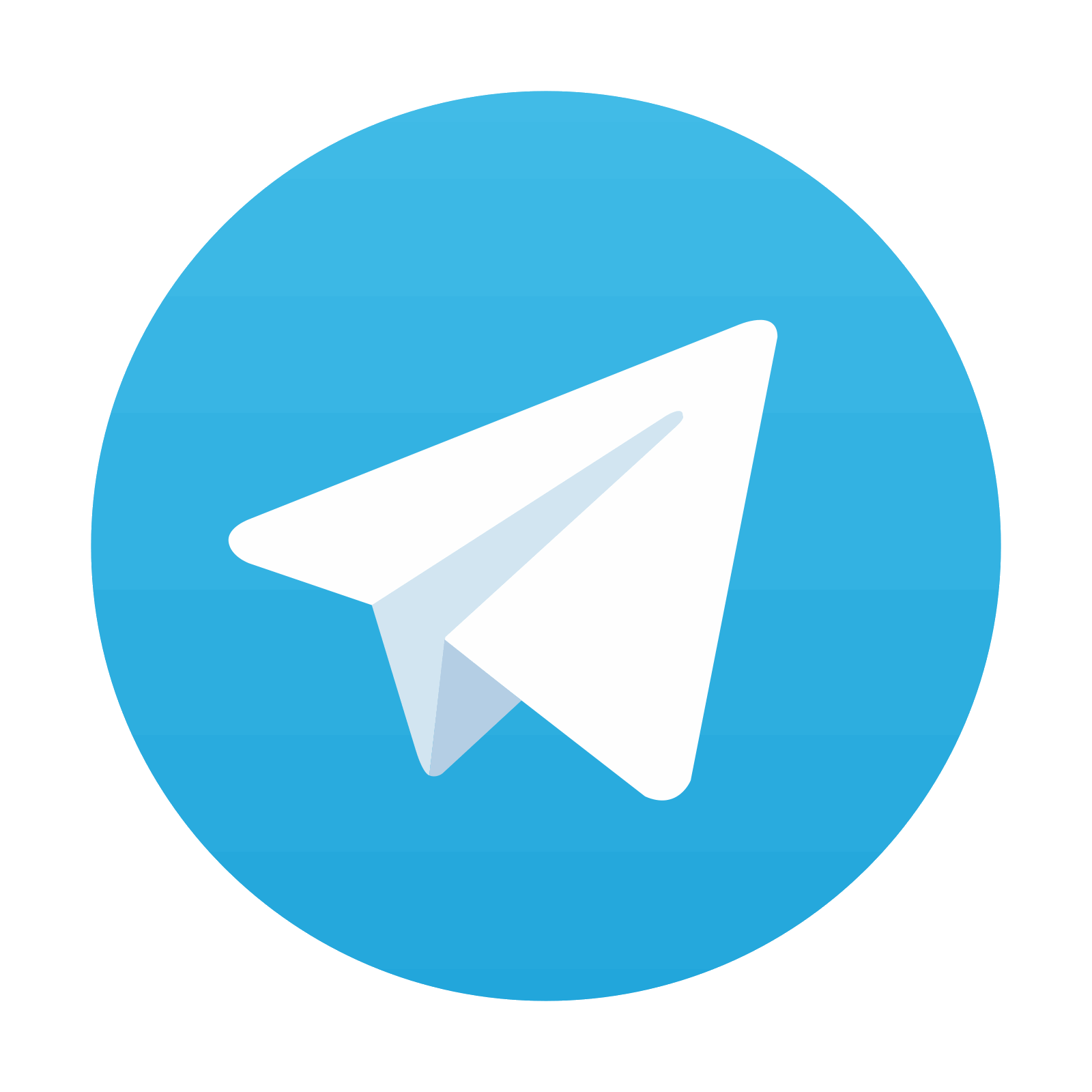