Thyroid Hormone Synthesis: Thyroid Iodide Transport
Nancy Carrasco
Iodine [or iodide (I–) in its ionized form] is an essential nutrient primarily because of the role it plays as an indispensable component of the thyroid hormones T3 and T4 [triiodothyronine and thyroxine (or tetraiodothyronine), respectively]. The thyroid hormones are iodothyronines, i.e., the result of two coupled iodotyrosines, and are the only iodine-containing hormones in vertebrates. Without iodine there is no biosynthesis of thyroid hormones. Therefore, thyroid function ultimately depends on an adequate supply of iodine to the gland. A remarkably efficient and specialized system has evolved in the thyroid that ensures that most of the ingested dietary I– (the only source of I–) is accumulated in the gland and thus made available for T3 and T4 biosynthesis. The significance of this becomes more apparent when one considers that I– is rather scarce in the environment. Endemic goiter and cretinism caused primarily by insufficient dietary supply of I– remain a major health problem in many parts of the world, affecting millions of people (1). This situation dramatizes the health value of I– as a nutrient and the consequences to society of its environmental scarcity.
Iodine was discovered in 1811 by Courtois, who isolated iodine from treating seaweed ash with sulfuric acid (2). The ability of the thyroid to concentrate I– was first reported as early as the 19th century (3,4). The thyroid gland was found to be capable of concentrating I– by a factor of >40 with respect to its concentration in the plasma under physiologic conditions. Hence the existence of a thyroid I– transporter was inferred, and some of its properties were elucidated over the years [see (5,6,7) for reviews]. Briefly, I– accumulation in the thyroid has long been shown to be an active transport process that occurs against the I– electrochemical gradient, stimulated by thyrotropin (TSH), and blocked by the well-known “classic” competitive inhibitors, the anions thiocyanate (SCN–) and perchlorate (ClO4–). Eventually, it was determined that the thyroid I– transporter is a Na+/I– symporter (NIS) (8,9,10,11,12), i.e., an intrinsic plasma membrane transport protein that couples the inward “downhill” translocation of Na+ to the inward “uphill” translocation of I– (Fig. 4A.1). The driving force for the process is the inwardly directed Na+ gradient generated by the Na+/K+ ATPase (Fig. 4A.1). The ability of the thyroid to accumulate I– via NIS has long provided the basis for diagnostic scintigraphic imaging of the thyroid with radioiodide, and has served as an effective means for administered radioiodide to target and destroy hyperfunctioning thyroid tissue, such as in Graves’ disease, or I–-transporting thyroid cancer cells. Therefore, the study of NIS is of great relevance to thyroid pathophysiology. Nevertheless, no molecular information on NIS was available until 1996, when after a decade-long search by numerous investigators, the cDNA encoding rat NIS was finally isolated by Dai et al. by expression cloning in Xenopus laevis oocytes (13). This development marked the beginning of the molecular characterization of NIS.
NIS mediates the first and key step in the process of supplying I– to the gland for thyroid hormone biosynthesis, i.e., the active transport of I– against its electrochemical gradient across the basolateral plasma membrane into the cytoplasm of the follicular cells (I– uptake) (Fig. 4A.1). NIS also mediates active I– transport in a few other tissues, including lactating breast, salivary glands, stomach, and small intestine (see later). Underscoring the significance of NIS for thyroid function, in a study on the ontogeny of various key proteins in thyroid tissues from human embryos and fetuses, Szinnai et al. (14) showed that NIS was the last of these proteins to be expressed, and that NIS function was not only the final step in thyroid differentiation but also the final step required for the onset of thyroid function in humans.
After reaching the cytosol, I– is passively translocated across the apical membrane into the colloid, located in the follicular lumen (I– efflux). I– efflux to the follicular lumen has been suggested to be mediated by a different plasma membrane transporter located on the apical side of the follicular cells, facing the colloid. The identity of this transporter has yet to be unequivocally established. Two different proteins, pendrin (15) and the protein formerly (and erroneously) known as the apical iodide transporter (AIT) (16), have been proposed to play the role of mediator of I– efflux. However, there is evidence that casts doubt on pendrin playing this role or being the only molecule to do so, and the protein formerly named AIT is now known to transport monocarboxylates, not I–, which conclusively rules it out as the mediator of I– efflux. It is possible that I– efflux is instead mediated by Cl– channels or transporters (Fig. 4A.1), but this step in thyroid hormone biosynthesis needs to be investigated further.
NIS research at the molecular level has become an exciting field in thyroidology. This chapter, after giving some historical perspective, focuses on the most recent such research, and on the impact it has on our understanding of thyroid pathophysiology and on a wide variety of basic and clinical fields, including structure and function of and mechanistic information on membrane transporters, cancer, gene transfer studies, and public health.
Molecular characterization of NIS
Identification of the NIS cDNA
A promising development in the search for the NIS molecule was the expression of ClO4–-sensitive Na+/I– symport activity
in X. laevis oocytes, by Vilijn et al., by microinjection of poly-A+ RNA isolated from FRTL-5 cells, a highly functional line of rat thyroid–derived cells (11). A 7-fold increase of I– accumulation was observed over background, 6 to 7 days after injection. Poly-A+ RNA was subsequently fractionated by sucrose gradient centrifugation, and fractions were assayed for their ability to induce I– accumulation in oocytes. The poly-A+ RNA encoding NIS was found in a fraction containing mRNAs 2.8 to 4.0 kb in length (11). Thus, in the absence of oligonucleotides based on protein sequence data or anti-NIS antibodies, the oocyte system was shown to be of value for the possible expression cloning of the cDNA that encodes NIS. After a few years, this cloning strategy was successful.
in X. laevis oocytes, by Vilijn et al., by microinjection of poly-A+ RNA isolated from FRTL-5 cells, a highly functional line of rat thyroid–derived cells (11). A 7-fold increase of I– accumulation was observed over background, 6 to 7 days after injection. Poly-A+ RNA was subsequently fractionated by sucrose gradient centrifugation, and fractions were assayed for their ability to induce I– accumulation in oocytes. The poly-A+ RNA encoding NIS was found in a fraction containing mRNAs 2.8 to 4.0 kb in length (11). Thus, in the absence of oligonucleotides based on protein sequence data or anti-NIS antibodies, the oocyte system was shown to be of value for the possible expression cloning of the cDNA that encodes NIS. After a few years, this cloning strategy was successful.
Dai et al. (13) generated several cDNA libraries from poly-A+ RNA of FRTL-5 cells, and subjected them to expression screening in X. laevis oocytes. The library was size-fractionated and the fraction containing inserts from 2.5 to 4.5 kb was screened because the poly-A+ RNA encoding NIS was found in a fraction containing messages of 2.8 to 4.0 kb in length. The expression cloning of NIS was carried out by measuring ClO4–-sensitive Na+/I– symport activity in oocytes microinjected with cRNAs made in vitro from pools containing decreasing numbers of cDNA clones. The cloning of NIS marked the beginning of its molecular characterization, as discussed below.
Primary Sequence and Secondary Structure Model of NIS
The complete nucleotide sequence of the cloned NIS cDNA and the deduced amino acid sequence are presented in reference (13). Beginning with Met at position 1, a long open reading frame codes for a protein of 618 amino acids (relative molecular mass 65,196). The hydropathic profile and initial secondary structure predictions of the protein led Dai et al. (13,17) to suggest an intrinsic membrane protein with 12 putative transmembrane segments (TMS). However, this model has subsequently been revised upon extensive experimental testing, as detailed below. The NH2 terminus was originally placed on the cytoplasmic side, given the absence of a signal sequence. The COOH terminus, which was also predicted to be on the cytoplasmic side, was found to contain a large hydrophilic region of ∼70 amino acids. Levy et al. demonstrated experimentally the intracellular orientation of the COOH terminus by showing that an anti-COOH terminus antibody binds to its epitope only after cell permeabilization (18). Three potential Asn-glycosylation sites were identified in the deduced amino acid sequence at positions 225, 485, and 497. The first was located in a predicted intracellular hydrophilic loop, while the last two were located in the last hydrophilic loop, a segment predicted to be on the extracellular face of the membrane.
Levy et al. (19) obtained conclusive evidence showing that, contrary to previous suggestions (20), neither partial nor total lack of N-linked glycosylation impairs activity, stability, or targeting of NIS. Using site-directed mutagenesis, Levy et al. (19) substituted both separately and simultaneously the Asn residues (amino acids 225, 485, and 497) with Gln in all three putative N-linked glycosylation consensus sequences of NIS, and assessed the effects of the mutations. All mutants were active and displayed 50% to 100% of wild-type NIS activity, including the completely non-glycosylated triple mutant. They observed that the half-life of non-glycosylated NIS was similar to wild-type NIS and that the Km value for I– (∼20 to 30 μM) in non-glycosylated NIS was virtually identical to that of wild-type NIS. These findings demonstrate that, to a considerable extent, function, targeting, and stability of NIS are present even in the total absence of N-linked glycosylation. Therefore, a bacterial expression system, in which no N-linked glycosylation occurs, might be used to overproduce NIS for structural studies.
Levy et al. (19) also demonstrated that the putative N-linked glycosylation site at N225, which had originally been predicted to face intracellularly, is indeed glycosylated. Therefore, it is now clear that the hydrophilic loop that contains this sequence faces the extracellular milieu rather than the cytosol. They have proposed a 13-TMS model to be the most likely secondary structure for NIS (Fig. 4A.2). In contrast to the original model, in which the NH2 terminus was predicted to face the cytosol on account of the lack of a signal sequence in NIS, in the current model, both the NH2 terminus and the hydrophilic loop containing N225 are predicted to be on the extracellular side, and the COOH terminus faces the cytosol.
Levy et al. (19) subsequently demonstrated unequivocally that the NH2 terminus faces the external milieu, as proposed in the current model. This conclusion was reached using two independent experimental approaches. First, these authors introduced a FLAG epitope at the NH2 terminus. COS cells transfected with FLAG-containing NIS displayed undistinguishable I– uptake accumulation from that of COS cells transfected with wild-type NIS. Immunofluorescence experiments revealed positive immunoreactivity with anti-Flag Ab in non-permeabilized COS cells transfected with FLAG-containing NIS. Positive immunoreactivity in non-permeabilized cells indicates that the NH2 terminus faces externally. In contrast, immunoreactivity using anti-COOH Ab requires permeabilization because the COOH terminus faces the cytosol (18). The second technique took advantage of a previous observation that non-glycosylated NIS is active. The N-linked glycosylation amino acid sequence NNSS was introduced into the NH2 terminus of non-glycosylated NIS (21). They observed glycosylation of NIS at the NH2 terminus upon transfection of NNSS-containing NIS into COS cells, thus proving that the NH2 terminus faces the lumen of the endoplasmic reticulum during biosynthesis and therefore faces the external milieu upon reaching the plasma membrane.
NIS, the product of the SLC5A5 gene, is a member of solute carrier family 5 (SLC5), which also includes – among many other proteins that mediate key physiologic transport processes – Na+/glucose co-transporter 1 (SGLT1) (22). The current 13-TMS model for NIS has been confirmed with the determination, by Faham et al. (23), of the structure of vSGLT, the Vibrio parahaemolyticus Na+/galactose transporter, with which NIS shares significant identity (27%) and homology (58%). vSGLT is the bacterial homologue of the human SGLT1.
NIS Mechanism and Stoichiometry
Eskandari et al. (24) have examined the mechanism, stoichiometry, and specificity of NIS by means of electrophysiologic, tracer uptake, and electron microscopic methods in X. laevis oocytes expressing NIS. These authors obtained electrophysiologic recordings using the two-microelectrode voltage clamp technique. They showed that an inward steady-state current (i.e., a net influx of positive charge) is generated in NIS-expressing oocytes upon addition of I– to the bathing medium, leading to depolarization of the membrane. Since the recorded current is attributable to NIS activity, this observation confirms that NIS activity is electrogenic. Simultaneous measurements of tracer fluxes and currents revealed that two Na+ ions are transported with one anion, demonstrating unequivocally a 2:1 Na+/I– stoichiometry. Therefore, the observed inward steady-state current is due to a net influx of Na+ ions.
Eskandari et al. (24) determined that the turnover rate of NIS is ∼36 s-1, and reported that expression of NIS in oocytes led to a ∼2.5-fold increase in the density of plasma membrane protoplasmic face intramembrane particles, as ascertained by freeze-fracture electron microscopy. This is the first direct electron microscopy visualization of ostensible NIS molecules present in the oocyte plasma membrane.
Specificity of NIS: NIS Transports Different Substrates with Different Stoichiometries
Similar steady-state inward currents were generated by a wide variety of anions in addition to I– (including ClO3–, SCN–, SeCN–, NO3–, Br–, BF4–, IO4–, and BrO3–), indicating that these anions are also transported by NIS. However, ClO4–, the most widely characterized inhibitor of thyroidal I– uptake, was surprisingly found not to generate a current, strongly suggesting that it was not transported (24). Yoshida et al. (25) have reported, similarly, that ClO4– did not induce an inward current in Chinese hamster ovary (CHO) cells stably expressing NIS, as measured using the whole-cell patch clamp technique. The most likely interpretation of these observations, at the time, seemed to be that ClO4– was not transported by NIS, although the unlikely possibility that ClO4– was translocated by NIS with an electroneutral 1:1 Na+/ClO4– stoichiometry could not be ruled out. To unequivocally elucidate whether ClO4– is translocated or not by NIS, flux experiments needed to be performed with ClO4– labeled with 36Cl; however, the unavailability of 36ClO4– made such experiments impossible. Yoshida et al. (26) thereafter showed that ClO4– elicited no change in the membrane current in the highly functional rat thyroid cell line FRTL-5, as revealed by the whole-cell patch-clamp technique, consistent with the notion that ClO4– was not transported into FRLT-5 cells and supporting both their previous observations in CHO cells (25) and supporting the results of Eskandari et al. (24) in oocytes. All these results, taken together, led researchers to conclude that ClO4– was a potent inhibitor of NIS that acted as a blocker, not a substrate.
However, a study carried out by Dohán et al. (27) using a bioassay has conclusively – and surprisingly – demonstrated that ClO4– in fact is actively translocated by NIS after all. These authors employed an indirect approach in a polarized in vitro bicameral model system. The model recapitulated a polarized epithelial monolayer with a basolateral and an apical surface. In the model, a monolayer of polarized epithelial Mardin-Darby canine kidney (MDCK) cells stably expressing exogenous NIS (engineered to be targeted apically in these cells) separated the lower (basolateral) from the upper (apical) chamber. The authors added I– to the apical chamber and followed the change in I– concentration in both chambers over time. I– was rapidly transported from the apical to the basolateral chamber, and the gradient generated was maintained for >6 hours. When I– and ClO4– were simultaneously added to the apical chamber, I– was also translocated to the basolateral chamber, except that transport occurred after a considerable delay (>1 hour), suggesting that the ClO4– concentration had to decrease beyond a certain point before NIS could start translocating I–.
Moreover, to investigate whether ClO4– reached the opposite chamber, ClO4– alone (without I–) was added to the apical chamber, the basolateral chamber, or both. Knowing that NIS was exclusively expressed apically in these cells, the authors took aliquots from the apical or basolateral chamber and added them to separate NIS-expressing MDCK cells to assess whether the aliquots would inhibit I– transport, indicating that the aliquots contained ClO4–. The authors found that only aliquots from the basolateral chamber were markedly inhibitory, even when ClO4– was only added to the apical chamber, demonstrating that ClO4– was actively translocated to the basolateral chamber by NIS (27).
Dohán et al. extended their in vitro observations by injecting 131I– or 131I–/ClO4– into lactating dams but not into their pups and by showing that thyroidal I– uptake in both dams and pups was markedly lower in the ClO4–-treated animals than in the controls. Furthermore, to investigate whether ClO4– not only inhibited NIS function but also was actually translocated by NIS into the milk, the authors injected ClO4– into lactating dams and tested whether milk samples inhibited NIS-mediated I– transport in MDCK cells expressing NIS. They observed that only milk samples from animals that received ClO4– inhibited I– transport. In conclusion, it is clear that ClO4– is not a non-transported blocker of NIS. Instead, it reaches the milk by NIS-mediated active transport, not by passive diffusion.
It is now evident that ClO4– elicited no currents in electrophysiology experiments because Na+-dependent ClO4– transport is electroneutral and, therefore, its kinetic analysis could only be carried out by direct substrate flux measurements (which, as indicated above, are not feasible). Thus, Dohán et al. (27) instead analyzed the kinetic parameters of NIS-mediated transport of a structurally similar anion, perrhenate (ReO4–), taking advantage of the higher specific activity of the radioisotope 186ReO4–. The initial rates of NIS-mediated, Na+-dependent ReO4– transport yielded a hyperbolic curve indicative of an electroneutral stoichiometry, strongly suggesting that the NIS-mediated Na+/ClO4– transport stoichiometry is also electroneutral. This finding stands in stark contrast to the electrogenic 2:1 Na+/anion stoichiometry observed with most other NIS substrate anions, demonstrating that NIS translocates different substrates with different stoichiometries, a remarkable property that had not previously been discovered in any transporter. A simple mathematical model accurately predicts the degree of competition between ClO4– and I–, and that ClO4– is transported with a Vmax 3 times lower and a backflow rate 10 times lower than that of I– (27). The results of Dohán et al. have been confirmed by Tran et al. (28), who showed, using a sensitive chromatography–electrospray ionization-tandem mass spectrometry method, that ClO4– is actively transported in FRTL-5 cells.
Impact on Public Health
The above-cited findings on NIS-mediated transport of ClO4–, in addition to their significance in the area of structure/function of plasma membrane transporters regarding the existence of different stoichiometries for different substrates, are also having a considerable impact on an ongoing debate on the public health effects of ClO4– pollution. Numerous sources of drinking water in the United States are contaminated with ClO4– as a result of industrial production of the anion, which is used as a propellant and as an explosive. As a result, low-level exposure to ClO4– is common in the U.S. population and has been associated with increased serum TSH and decreased T4 levels in women with a low iodine status (29). The demonstration that NIS actively transports ClO4– and mediates its translocation to the milk indicates that ClO4– may pose a health risk, particularly to nursing newborns, although recent studies have not shown an effect of low levels of ClO4– on thyroid function in first trimester pregnant women (including those with a urinary iodine less than 100 μg/L) (30,31) and in neonates of nursing mothers (32). Exposure of a nursing mother to high levels of ClO4– would lead to both lower I– and higher ClO4– in the milk, ultimately compromising the newborn’s own thyroidal I– uptake and thyroid hormone biosynthesis. These effects would seriously impair neurologic and overall physical development in utero.
Regulation of NIS
Regulation of NIS Transcription
Three different transcription factors have been implicated in thyroid-specific gene transcription (33,34): (1) Thyroid transcription factor 1 (TTF-1), a homeodomain (HD)-containing protein present in the developing thyroid, lung, forebrain, and pituitary and in the adult thyroid and lung; (2) TTF-2, a forkhead protein detected in developing thyroid and anterior pituitary and in the adult thyroid; and (3) Pax8, a nuclear protein member of the murine family of paired-domain (PD) containing genes, present in the developing thyroid, kidney, and mid-brain boundary and in the adult thyroid and kidney. Specific combinations of these factors have been proposed to regulate transcription of the thyroid-specific proteins thyroglobulin (Tg) and thyroperoxidase (TPO) and also of the TSH receptor (TSHr).
Isolation of the cDNA Encoding Human NIS (hNIS) and Elucidation of the Genomic Organization of hNIS
The hNIS cDNA was identified on the expectation that hNIS would be highly homologous to rNIS. Using primers to the cDNA rNIS sequence (specifically rNIS nucleotide sequences 362 to 480 and 671 to 900), Smanik et al. (35) amplified a cDNA fragment of hNIS from human papillary thyroid carcinoma tissue by PCR, utilized this cDNA fragment to screen a human thyroid cDNA library, and isolated a single cDNA clone encoding hNIS. The nucleotide sequence of hNIS revealed an open reading frame of 1,929 nucleotides, which encodes a protein of 643 amino acids. hNIS exhibits 84% identity and 93% similarity to rNIS. hNIS differs from rNIS only on account of a five amino acid insertion between the last two hydrophobic domains (amino acids 485 to 488 and 499) and a 20 amino acid insertion in the COOH terminus (amino acids 618 to 637). Subsequently, Smanik et al. (36) examined the expression, exon–intron organization, and chromosome mapping of hNIS. Fifteen exons encoding hNIS were found to be interrupted by 14 introns (Fig. 4A.3), and the hNIS gene was mapped to chromosome 19p. The human NIS promoter has been sequenced by three different groups (37,38,39).
Analysis of the Rat and Human NIS Promoters
It has long been established that TSH stimulates NIS activity via cAMP (40,20,41) and more recently that it upregulates NIS
mRNA levels (42). However, to fully understand these mechanisms it is necessary to study the transcriptional regulation of NIS. As for rat NIS, Endo et al. (43) localized a TTF-1 binding site between -245 and -230 bp, in the proximal rNIS promoter (2 kb), that confers thyroid-specific transcription but only exerts a modest effect. The same group (44) subsequently identified, in the 5′-flaking region between -1,968 and +1 bp, a novel TSH-responsive element (TRE) between -420 and -385 bp upstream of the TTF-1 site in the rNIS promoter, that upregulated 2- to 3-fold NIS expression. The TSH effect is cAMP-mediated and thyroid-specific. They showed that the protein that binds this site is different from TTF-1, TTF-2, Pax8, or other known transcription factors, and the authors named the putative binding protein in the TRE site NTF-1 (NIS TSH-responsive factor 1). However, as the TSH upregulation of NIS via this TRE site is lower than the regulation that the same group reported previously (∼6-fold) (42), they suggested that other transcription binding sequences may be present upstream from the 1968 bp region they studied.
mRNA levels (42). However, to fully understand these mechanisms it is necessary to study the transcriptional regulation of NIS. As for rat NIS, Endo et al. (43) localized a TTF-1 binding site between -245 and -230 bp, in the proximal rNIS promoter (2 kb), that confers thyroid-specific transcription but only exerts a modest effect. The same group (44) subsequently identified, in the 5′-flaking region between -1,968 and +1 bp, a novel TSH-responsive element (TRE) between -420 and -385 bp upstream of the TTF-1 site in the rNIS promoter, that upregulated 2- to 3-fold NIS expression. The TSH effect is cAMP-mediated and thyroid-specific. They showed that the protein that binds this site is different from TTF-1, TTF-2, Pax8, or other known transcription factors, and the authors named the putative binding protein in the TRE site NTF-1 (NIS TSH-responsive factor 1). However, as the TSH upregulation of NIS via this TRE site is lower than the regulation that the same group reported previously (∼6-fold) (42), they suggested that other transcription binding sequences may be present upstream from the 1968 bp region they studied.
Ohno et al. (45) showed that the rNIS regulatory region contains an enhancer located between -2,264 and -2,495 bp that recapitulates the most relevant aspects of NIS regulation (Fig. 4A.4). This enhancer mediates thyroid-specific gene expression by the interaction of Pax8 with a novel cAMP-dependent pathway. The NIS upstream enhancer (NUE) stimulates transcription in a thyroid-specific and cAMP-dependent manner. NUE contains: Two Pax8 binding sites, two TTF-1 binding sites that have no effect on rNIS transcription, and a degenerate CRE (cAMP-responsive element) sequence (5′-TGACGCA-3′), which is important for NUE transcriptional activity (Fig. 4A.4). In NUE, both Pax8 and the CRE-like binding factor act synergistically to obtain full TSH/cAMP-dependent transcription. However, this enhancer is also able to mediate cAMP-dependent transcription by a novel PKA-independent mechanism (45). Transcriptional regulation of the Tg, TPO, and TSHr genes by TSH/forskolin is mediated by cAMP. However, no CRE sequences have been identified for any of these, except the TSHr gene. Thus, the novel PKA-independent mechanism reported by Ohno et al. (45) is highly significant, as it established a direct relationship between thyroid-specific transcription factors and the TSH/cAMP
regulation in rNIS. This picture clearly separated rNIS gene regulation from that of true thyroid-restricted genes (Tg and TPO) and also from TSHr, a notion consistent with the fact that NIS is not exclusively expressed in the thyroid gland. Subsequently, Chun et al. (46) demonstrated that the CRE-like element within NUE interacts with different b-Zip molecules, such as Fos/Jun and CREB/ATF. Although the CRE-like element plays a central role in mediating cAMP-induced stimulation of enhancer activity, it requires the cooperation of at least one of the two Pax8 binding sites. Although the synergistic effect of Pax8 and the b-Zip molecules requires that they bind to adjacent sites in NUE, they do not appear to physically interact with each other directly.
regulation in rNIS. This picture clearly separated rNIS gene regulation from that of true thyroid-restricted genes (Tg and TPO) and also from TSHr, a notion consistent with the fact that NIS is not exclusively expressed in the thyroid gland. Subsequently, Chun et al. (46) demonstrated that the CRE-like element within NUE interacts with different b-Zip molecules, such as Fos/Jun and CREB/ATF. Although the CRE-like element plays a central role in mediating cAMP-induced stimulation of enhancer activity, it requires the cooperation of at least one of the two Pax8 binding sites. Although the synergistic effect of Pax8 and the b-Zip molecules requires that they bind to adjacent sites in NUE, they do not appear to physically interact with each other directly.
Regarding the human NIS gene, a thyroid-specific, TSH-responsive, far-upstream (-9,847 to -8,968) enhancer – highly homologous to the rat NUE – has been reported by two groups (47,48). It contains putative Pax8 and TTF-1 binding sites and a CRE-like sequence. The TTF-1 binding site is not required for full activity (Fig. 4A.4) (47,48).
Regulation of NIS in the Thyroid by TSH
TSH is the primary hormonal regulator of thyroid function overall, and has long been known to stimulate thyroidal I– accumulation. Most actions of TSH take place through activation of adenylate cyclase via the GTP binding protein Gα. This cascade of events is initiated by the interaction of TSH with its receptor on the basolateral membrane of the follicular cells. Early observations made prior to the isolation of the NIS cDNA suggested that TSH stimulation of I– accumulation results, at least in part, from the cAMP-mediated increased biosynthesis of NIS (40,41). Once the rat NIS cDNA was isolated (13) and anti-NIS Abs were generated, Levy et al. demonstrated in rats that NIS protein expression is upregulated by TSH in vivo (18). Consistent with these findings is a later observation by Uyttersprot et al. (49) that the expression of NIS mRNA in dog thyroid (∼3.9 kb) is dramatically upregulated by goitrogenic treatment (i.e., propylthiouracil (PTU) treatment, which leads to elevated TSH circulating levels in vivo).
Upregulation of thyroid NIS expression and I– uptake activity by TSH has been demonstrated not only in rats in vivo (18), but also in the rat thyroid–derived FRTL-5 cell line (41) and in human thyroid primary cultures (50,51). Marcocci et al. (52), Kogai et al. (42) and Ohno et al. (45) have all shown that TSH upregulates I– uptake activity by a cAMP-mediated increase in NIS transcription. After TSH withdrawal, a reduction of both intracellular cAMP levels and I– uptake activity is observed in FRTL-5 cells (41). This is a reversible process, as I– uptake activity can be restored either by TSH or agents that increase cAMP (41,45). To investigate NIS biogenesis, Levy et al. (18) carried out metabolic labeling and immunoprecipitation experiments in the presence of TSH, and observed that NIS is synthesized as a precursor (∼56 kDa) (19). After a 60-minute chase period, a broad, fully processed polypeptide band (∼90 kDa) also became apparent.
Kaminsky et al. (40) made the surprising observation that I– uptake activity is present in membrane vesicles (MVs) prepared from FRTL-5 cells that, when intact, had completely lost I– uptake activity due to prolonged TSH deprivation (Fig. 4A.5). This suggests that mechanisms other than transcriptional may also operate to regulate NIS activity in response to TSH.
Kogai et al. (50) have shown that TSH markedly stimulates NIS mRNA and protein levels in both monolayer and follicle-forming human primary culture thyrocytes, whereas significant stimulation of I– uptake is observed only in follicles. These interesting observations indicate that, in addition to TSH stimulation, cell polarization and spatial organization are also crucial for proper NIS activity, and suggest that NIS may be regulated by such posttranscriptional events as subcellular distribution. Riedel et al. (53) have demonstrated conclusively by immunoblot analysis that NIS is present in FRTL-5 cells as late as 10 days after TSH withdrawal and that de novo NIS biosynthesis requires TSH (53). Therefore, it is clear that any NIS molecules detected in TSH-deprived FRTL-5 cells had to be synthesized prior to TSH withdrawal. This is consistent with NIS being a protein with an exceptionally long half-life, as suggested by Kogai et al. (42) and Paire et al. (20). Riedel et al. (53) determined by pulse-chase analysis that the NIS half-life is ∼5 days in the presence of and ∼3 days in the absence of TSH. Even though the NIS half-life in the absence of TSH is 40% shorter than in the presence of the hormone, it is still sufficiently long to account for the persistence of significant I– uptake activity in MV from cells deprived of TSH (53).
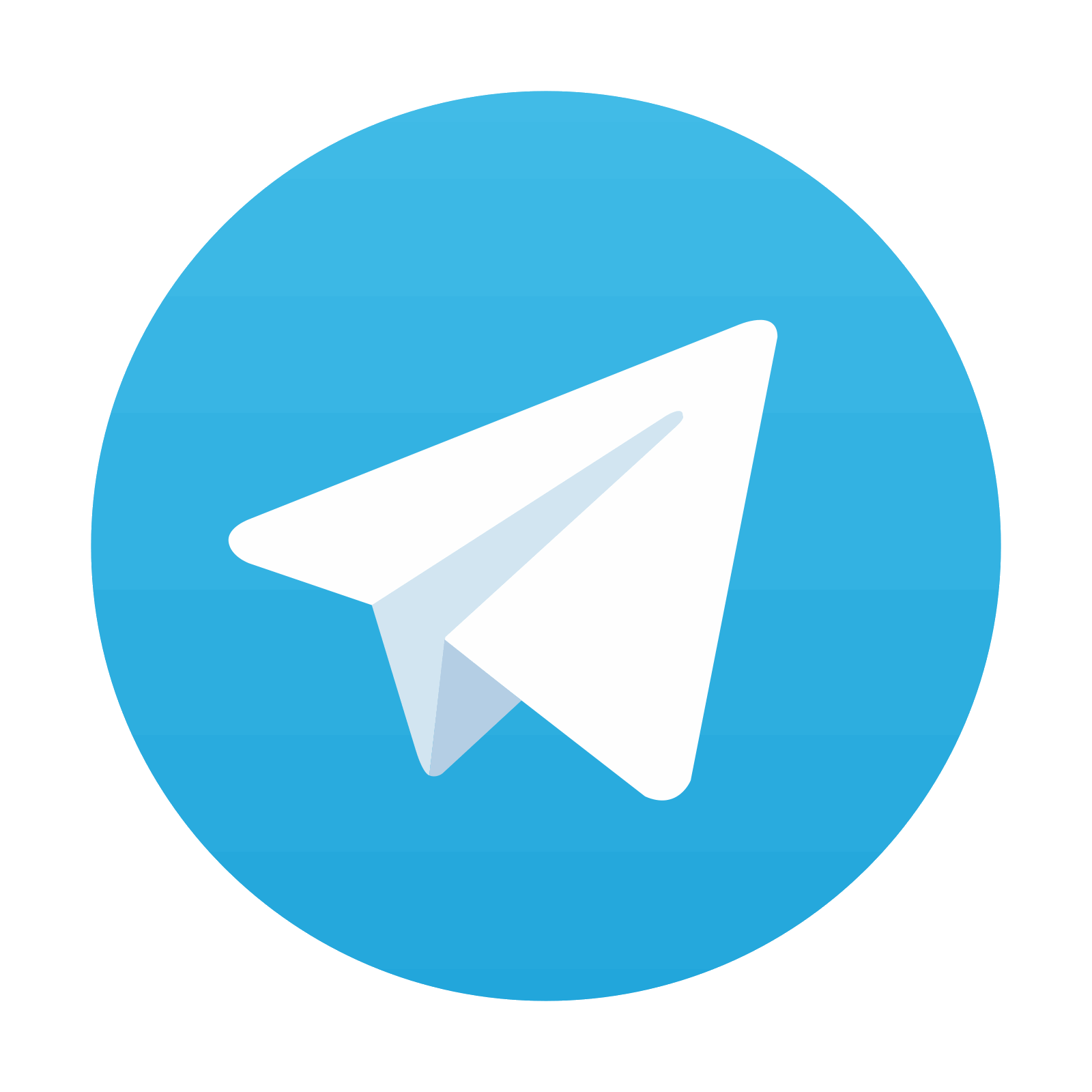
Stay updated, free articles. Join our Telegram channel
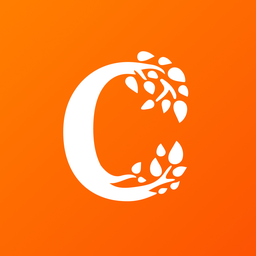
Full access? Get Clinical Tree
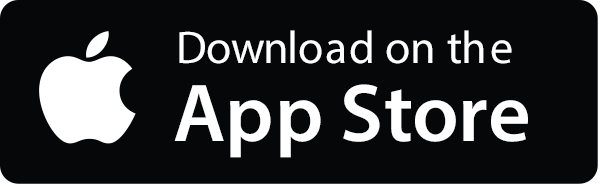
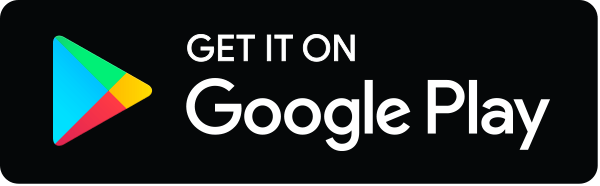
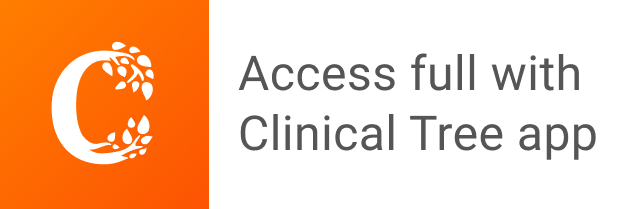