Thyroid Hormone Structure–Function Relationships
Stephen D. Ayers
John D. Baxter
Paul Webb
The parental form of thyroid hormone (thyroxine, T4) is subjected to multiple metabolic modifications that alter its activity. Analyses of the activities of T4 metabolites have been performed during the last century by administration to animals and utilization of cell-based and reporter gene assays. Early studies demonstrated that selective deiodination of T4 to produce triiodothyronine (T3) yields the major active version of the hormone (1,2). While some modifications of the T4 alanine side chain can also yield active hormones, most other enzymatic modifications of T4 and T3 produce inactive derivatives. Numerous studies with synthetic thyromimetics have defined the essential structural features of active thyroid hormones, and have provided a wealth of structure–activity relationship (SAR) data. Nearly all the actions of thyroid hormones are mediated by two closely related nuclear thyroid hormone receptors (TRs α and β) that are conditional transcription factors. X-ray crystallographic studies of TR ligand–binding domains (LBDs) along with supplementary biochemical, biophysical, and computational approaches have provided a detailed understanding of the molecular basis of hormone–receptor interactions, and clear explanations for the effects of particular chemical modifications upon ligand activity. This information, in parallel with advances in the medicinal chemistry of thyromimetics, has facilitated the development of synthetic TR antagonists and selective TR modulators (STRMs), which mimic the desirable aspects of hyperthyroidism (specifically, reduced serum lipids and weight loss) while avoiding deleterious effects (atrial arrhythmias, bone loss, and others) (3). The characterization of TR interactions with these ligands has also provided valuable new insights into the mechanisms of TR ligand recognition. While most actions of thyroid hormones are mediated by TRs, they also interact with other proteins that regulate their availability to target tissues, and bind to cell surface or intracellular proteins that could constitute alternate hormone signaling systems. In this chapter, we consider SARs of thyroid hormones and how their chemical composition influences interactions with TRs and other proteins.
Thyroid Hormone Structure, Modifications, and Activity
As described in Chapter 2, thyroid hormone is synthesized in the thyroid gland in a complex, stepwise process in which
thyroglobulin tyrosine residues are converted to iodotyrosine by the addition of inorganic iodine, covalently linked to form an iodinated diphenyl ether, and liberated from the parental protein into the circulation. This process produces the major form of secreted thyroid hormone, thyroxine (T4; 3′,5′,3,5-tetraiodo-L-thyronine, Fig. 9.1). The unique structural properties of this compound and its metabolites allow it to exert its biologic effects through specific protein interactions.
thyroglobulin tyrosine residues are converted to iodotyrosine by the addition of inorganic iodine, covalently linked to form an iodinated diphenyl ether, and liberated from the parental protein into the circulation. This process produces the major form of secreted thyroid hormone, thyroxine (T4; 3′,5′,3,5-tetraiodo-L-thyronine, Fig. 9.1). The unique structural properties of this compound and its metabolites allow it to exert its biologic effects through specific protein interactions.
The structural hallmark of all thyroid hormones is the thyronine nucleus, a diphenyl ether in which the two planar phenyl groups are oriented at an angle of 120 degrees (Fig. 9.1). T4 contains four bulky iodine substituents at the 3,5,3′ and 5′ positions, which hinder rotational flexibility and constrain the two rings to an almost perpendicular conformation. The outer ring also contains a 4′ hydroxyl group. The inner ring contains a side chain with the structure of alanine at the 1 position, which is usually zwitterionic (i.e., net positive charge at the amine group and net negative charge at carboxylic oxygen atoms) in solution at normal physiologic pH.
T4 is activated by selective deiodination (Fig. 9.2). Removal of the 5′ iodine group by deiodinase 1 or 2 (DIO1, DIO2) produces the major active thyroid hormone, T3; 3′,3,5-triiodo-L-thyronine) (4). T3 is normally present at relatively low levels in the circulation, where differences in rates of hormone secretion by the thyroid gland and longer serum half-life of T4 result in a serum ratio of T4/T3 of more than 20:1 (5). Selective deiodination of T4 is tightly regulated at the level of DIO2 expression in peripheral tissues. For example, circulating bile acids activate a G-coupled receptor (TGR5) that is expressed primarily in brown adipose tissue resulting in the induction of DIO2, increased T3 production, and induction of a T3 responsive gene, uncoupling protein 1 (UCP1), which promotes dissipation of the mitochondrial proton gradient and heat production (6). T3 is approximately five times more potent than T4. Catalytic removal of the 5′ iodine reduces steric bulk at the 5′ position of the outer ring, but does not alter rotational flexibility of the rings or overall conformation of the hormone relative to T4. As a result of the differences in pKa of the 4′-OH in T3 and T4 (8.47 and 6.73, respectively), the 3′-OH of T3 is significantly less ionized than T4 at physiologic pH (about 10% vs. 80%).
T4 and T3 are subjected to other deiodination steps and modifications that inactivate the hormone (Fig. 9.2). DIO3 acts on T4 and T3 to remove the iodine at the 5 position of the inner thyronine ring and produces reverse T3 (rT3) (7) and T2, respectively. rT3 is present at relatively high levels in the circulation, but binds TRs with an affinity that is several orders of magnitude lower than T4 or T3 and also exhibits reduced activity relative to both major forms of thyroid hormone. T2 is completely inactive upon TRs but may exert some metabolic effects on cells via interactions with other intracellular proteins (see section on Thyroid Hormone Interactions with Alternate Binding Proteins).
Removal of the inner ring iodine relieves constraints against rotational flexibility between rings and permits rotational freedom of the outer ring. Other de-iodinated derivatives (T1, T0) are detectable but appear completely inactive, at least as judged by their capacity to bind and activate classical TRs. Sulfation and glucuronidation are the two most frequently observed alternate thyroid hormone modifications. End products of these substitutions, T3S and T3Glu, are inactive and are readily excreted (8).
Removal of the inner ring iodine relieves constraints against rotational flexibility between rings and permits rotational freedom of the outer ring. Other de-iodinated derivatives (T1, T0) are detectable but appear completely inactive, at least as judged by their capacity to bind and activate classical TRs. Sulfation and glucuronidation are the two most frequently observed alternate thyroid hormone modifications. End products of these substitutions, T3S and T3Glu, are inactive and are readily excreted (8).
Alterations of the alanine side chain can produce active thyroid hormones. T3 and T4 are converted to Triac (tri-iodothyroacetic acid) and Tetrac (tetra-iodothyroacetic acid), respectively, by L-amino acid oxidase and thyroid hormone aminotransferase (9). Triac is not present at high levels in the circulation, but appears to represent a significant fraction of liver thyroid hormone content and acts as a potent full TR agonist with slightly higher affinity for TRβ versus TRα. Like the relationship between T4 and T3, Tetrac exhibits reduced potency relative to Triac.
Thyronamines are another class of active thyroid hormone metabolites, putatively derived through respective deiodination and decarboxylation of thyroxine by deiodinases and the non-selective aromatic amino acid decarboxylase to form 3-iodothyronamine (T1AM) and thyronamine (T0AM) (10) (Fig. 9.2). These products have been detected in circulation and elicit potent physiologic effects, despite their uncharacteristic lack of iodine substituents, including reductions in body temperature and heart rate (11,12). The distinct structure of these compounds is the likely source of their unique physiologic activities. Although they are completely inactive upon classical TRs, they have been shown to activate G-protein–coupled protein receptors on the cell surface, including trace amine activated receptor 1 (TAAR1) (10,13,14) and possibly, monoamine transporters in the brain including dopamine and norepinephrine. These novel regulatory compounds may provide an explanation for some of the physiologic effects of thyroid states and could potentially serve as the basis for treatments of pathologic hyperthermia and other conditions.
Synthetic Thyroid Hormones
The development of synthetic thyroid hormone analogs is integrally linked to the understanding of thyroid physiology, and knowledge in this area has led to new ideas for the treatment of thyroid diseases and related conditions. In 1915, Edward Kendall isolated thyroid hormone and demonstrated that it was responsible for the physiologic activities of the thyroid gland. In the following years, T4 was found to be the secreted hormone responsible for thyroid gland effects (15,16,17). Later experiments with radiolabeled T4 suggested that it was readily metabolized to T3, and experiments with synthetic T3 confirmed that this form of thyroid hormone was primarily responsible for thyroidal effects (1,2,18,19,20,21). The experimental synthesis of related compounds revealed that certain analogs of T4 also exhibited similar activity (22).
The relative simplicity of thyroxine contributed to the synthesis of analogous compounds with similar activity (“thyromimetics”) and became one of the earliest targets of SAR studies (23,24,25). Though early studies relied on the rudimentary biologic assays available at that time, they revealed the key structural elements that defined thyroid hormone activity and the considerable structural malleability within this framework, exemplified by the wide range of thyromimetics shown in Fig. 9.3.
Active thyroid hormone derivatives conform to some aspects of basic T3 structure, but there is a wide degree of variability within this framework (24). Active thyromimetics contain two planar rings, separated by a short bridge. This oxygen bridge can be substituted with sulfur or even carbon, despite its lack of hydrogen bonding capacity relative to the oxygen bridge that characterizes the natural hormone (26). However, longer bridges reduce thyromimetic activity. Substituents are incorporated in the inner ring 3 and 5 positions and the outer ring 3′ and 5′ positions, and the composition of these groups have profound effects on a compound’s structure and activity. Iodine is not absolutely required; substitution of other halogens or alkyl groups, including methyl and propyl groups (27), resulted in compounds with similar activities (28,29,30,31). Thyromimetic activity is dependent on the size and conformation of substituted groups; with few exceptions, discussed in detail in sections “TR Antagonism and Flexibility in the TR-binding Pocket” and “Mechanisms of TR subtype Selectivity” below, very bulky substituents exhibited reduced activity and effective agonist ligands mostly displayed highly conserved volumes, in the order of 470 to 500 Å3. Charged ring substituents were also poorly tolerated. Substitution of the alanine side chain with groups such as propionate or benzoate resulted in activities similar to endogenous thyroid hormones (32,33,34). Recent studies have shown that the nature of some of these side-chain compositions are critical in determining isoform-specific activities (35), discussed in the section on Mechanisms of TR subtype Selectivity. Thus, thyroid hormone mimetics adhere to a specific set of biochemical rules to exhibit full activity; specifically, they must possess two planar rings with a short bridging group, hydrophobic substituents at the 3′, 5, and 3 positions and polar substituents at the position of the alanine core.
X-ray Structures of TRs with Natural and Synthetic Agonists
Discovery of thyroid hormone receptors in 1986 provided a new tool for investigations (36,37). Thyromimetic activity largely correlated with binding affinity for the binding sites of the receptors. Later SAR studies with more even elaborately derivatized compounds largely supported earlier work, described in the previous section, and consistently confirmed that key structural components of thyromimetics are needed for the high affinity of these compounds for their receptors.
Resolution of detailed atomic structures of TR LBDs in complex with agonists revealed the basis for TR activation by thyroid hormones and thyromimetics (38). Initial TR structures were of rat TRα LBD in complex with T3 as well as synthetic thyromimetics, including 3,5 dimethyl-3-isopropylthyronine (DIMIT) and others. Additional studies confirmed that conclusions derived from this structure applied to both TRs, irrespective of human or mammalian origin, and to T3 and other agonists.
The most surprising finding obtained from the TR structure related to the location of the hormone binding. Agonists were completely buried within the LBD, suggesting that the
domain folds around the hormone during the activation process, and that T3 and other agonists comprise part of the hydrophobic core of the protein (Fig. 9.4A). This was contrary to many prevailing models of the time; it was commonly thought that hormone would bind to an exposed surface site and exert allosteric effects on overall receptor conformation. Admission of ligand into the buried pocket is coupled to large increases in LBD stability; unliganded TR LBDs adopt a highly mobile molten globular state in solution, and ligand binding induces a tightly folded conformer (39). Thus, the protein folds tightly around hormone, and this process is accompanied by widespread conformational changes that propagate throughout the entire domain.
domain folds around the hormone during the activation process, and that T3 and other agonists comprise part of the hydrophobic core of the protein (Fig. 9.4A). This was contrary to many prevailing models of the time; it was commonly thought that hormone would bind to an exposed surface site and exert allosteric effects on overall receptor conformation. Admission of ligand into the buried pocket is coupled to large increases in LBD stability; unliganded TR LBDs adopt a highly mobile molten globular state in solution, and ligand binding induces a tightly folded conformer (39). Thus, the protein folds tightly around hormone, and this process is accompanied by widespread conformational changes that propagate throughout the entire domain.
The size, shape, and amino acid composition of the completed TR ligand–binding pocket is well-tailored to structural features of T3 and similar molecules. Pocket volume, defined with T3 and similar agonists, is approximately 600 Å3 and is a very tight fit for hormone or agonists (470 to 530 Å3) within the buried cleft (40). This may explains why TR agonist ligand volume is highly conserved and why very bulky substituents generally reduce the affinity of hormone for receptor. The pocket shape corresponds well to the perpendicular ring structure that is the major structural feature of active thyroid hormones and thyromimetics. This explains why diphenyl compounds which do not adopt a perpendicular structure are poor activators. The region of the pocket that accommodates the thyronine rings is comprised mostly of hydrophobic amino acids; there are extensive van der Waals interactions between ligand and pocket, and the three iodine atoms (or equivalent hydrophobic substituents) are accommodated within small, well-defined niches. The nature of this region of the pocket explains why hydrophobic thyronine ring substituents, but not charged
substituents are tolerated. The only polar contact in this region of the pocket is formed by the side chain of a buried histidine residue (His381 in TRα) hydrogen and the 4′ hydroxyl group on the outer thyronine ring; this interaction may lock the outer ring into place in the pocket and explain the comparatively rigid requirements for this group. The region of the pocket that envelopes the alanine side chain is highly polar; a cluster of positively charged arginine side chains forms a loose network of hydrogen bonds with the negatively charged amino acid carboxylate. This region of the pocket contains significant amount of free space which tends to be occupied by loosely packed water molecules that interact with the charged groups of the alanine tail.
substituents are tolerated. The only polar contact in this region of the pocket is formed by the side chain of a buried histidine residue (His381 in TRα) hydrogen and the 4′ hydroxyl group on the outer thyronine ring; this interaction may lock the outer ring into place in the pocket and explain the comparatively rigid requirements for this group. The region of the pocket that envelopes the alanine side chain is highly polar; a cluster of positively charged arginine side chains forms a loose network of hydrogen bonds with the negatively charged amino acid carboxylate. This region of the pocket contains significant amount of free space which tends to be occupied by loosely packed water molecules that interact with the charged groups of the alanine tail.
The reasons that selective deiodination of T4 at the 5′ position produces a more active hormone, T3, can be easily understood within the context of TR structure (Fig. 9.4). Agonist ligands promote packing of amphipathic carboxy-terminal helix 12 (Fig. 9.4A, bold) against a scaffold comprised of helices 3 and 5 on the surface of the domain (Fig. 9.4B and C). This event results in the formation a coactivator-binding surface, activation function 2 (AF-2), and partly occludes a corepressor-binding site which is presumably exposed and available for corepressor interaction in the absence of hormone. Thus, T3 alters H12 position to facilitate exchange of coactivators for corepressors. Analysis of hormone–receptor contacts reveals that the 5′ region of the T3 outer thyronine ring packs closely against the inner hydrophobic surface of H12 to lock it into the active configuration (41). Thus, removal of the 5′ iodine group is required for tight packing of H12 against the domain.
Lessons about TR Hormone Binding from Natural Mutants
Knowledge of SARs of thyroid hormone action was also informed by inherited mutations of the TRβ LBD, which are the most frequent causes of resistance to thyroid hormone (RTH), reviewed in Chapter 58. The disease is characterized by defective feedback inhibition of TSH production through the hypothalamic–pituitary–thyroid axis, elevated thyroid hormone levels, and the occasional presentation as possible thyrotoxicosis, owing to an elevated heart rate, which is mediated by TRα (42,43). Analogs with increased capacity to activate TRβ, such as Triac, were effective in the treatment of several cases of RTH (44,45,46), because their selective activation of TRβ alleviated the symptoms of RTH, while averting the harmful effects of TRα activation on the heart (10).
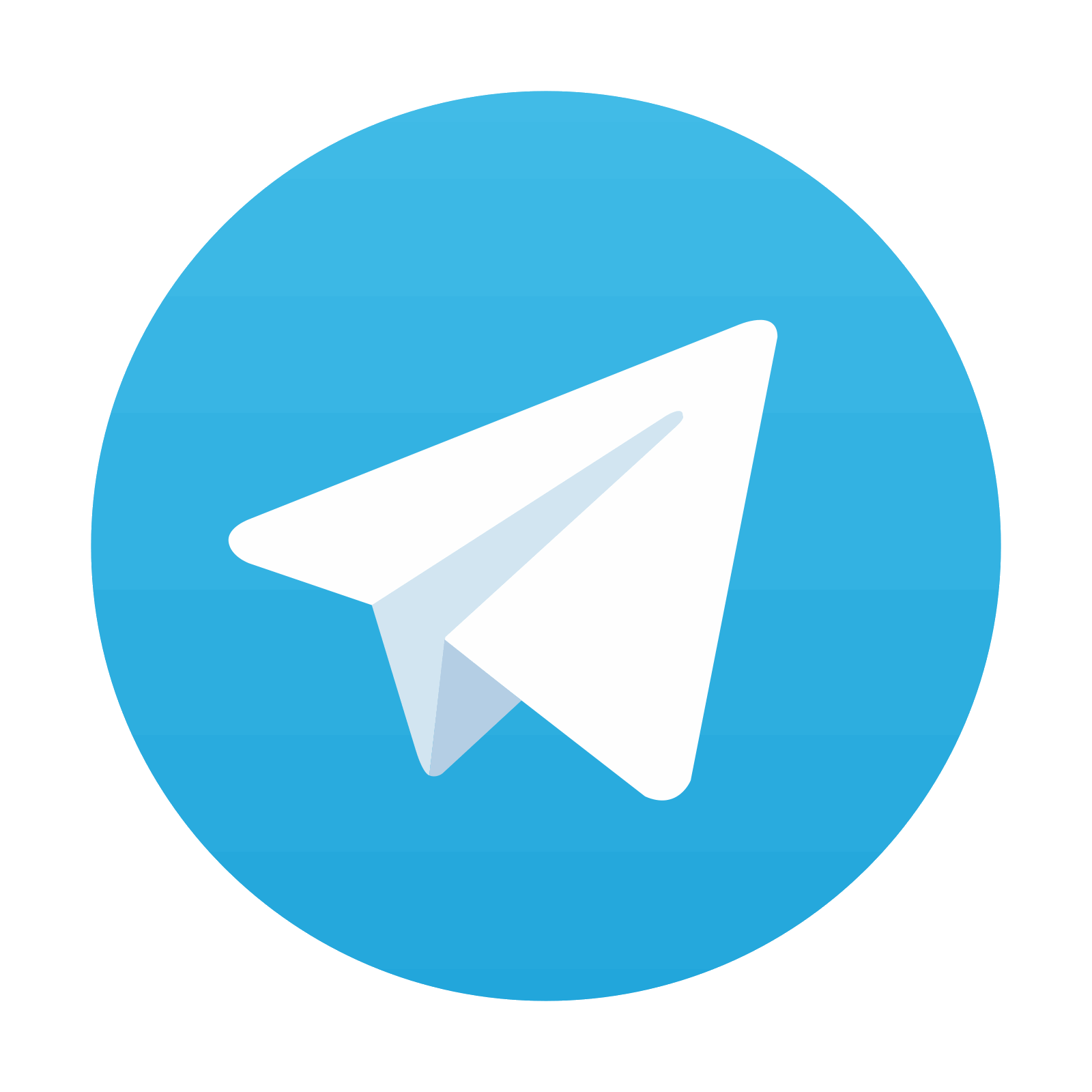
Stay updated, free articles. Join our Telegram channel
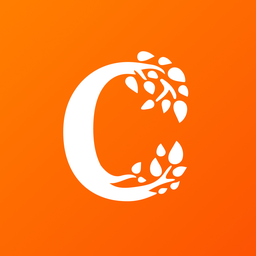
Full access? Get Clinical Tree
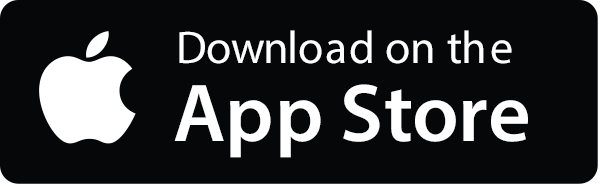
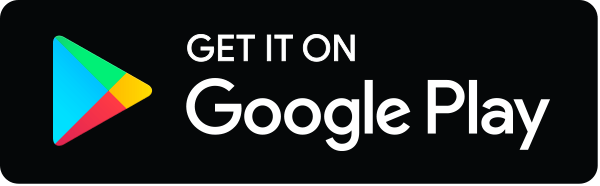