2.1
Introduction
Bone is a vital, dynamic connective tissue whose structure and composition reflect a balance among its major functions: provision of mechanical integrity for locomotion and for protection of vital organs, existence as a mineral reserve for supporting metabolic needs, and housing the tissue niches for hematopoiesis and skeletogenesis. Beginning with the observations of Galileo, it has been assumed that the shape and internal structure of bone are influenced by the mechanical loads associated with normal function. The 19th century saw active development of this concept, particularly with respect to the cross-sectional geometry of whole bones and to the structure of trabecular bone (see Refs. for a review). The most well-known of the published works from this time period is by Julius Wolff, who synthesized many others’ observations in postulating that the structure of trabecular bone is aligned with the principal stress directions that occur in this tissue during normal skeletal function . In this hypothesis, known by the misnomer “Wolff’s Law,” Wolff further proposed, as others before him had , that this alignment results from a self-regulating, functional adaptation process. Although errors in various components of Wolff’s writings have been identified , what is generally thought of today as Wolff’s Law is the overall concept that in bone, form follows function. This concept underlies much of the scientific investigation of relationships between bone structure and its mechanical and metabolic functions.
In maintaining these structure–function relationships, bone tissue is constantly being broken down and rebuilt in a process called remodeling . The cellular link between bone resorbing cells or osteoclasts, and bone forming cells or osteoblasts, is known as coupling. With age, remodeling tends to result in a negative bone balance; in that at each remodeling site, slightly less bone is deposited than is resorbed. This negative balance leads to osteopenia and osteoporosis, thus predisposing the bone to fracture during even minimal trauma. However, in normal states, the remodeling and modeling activities in bone serve to reduce bone mass where the mechanical demands of the skeleton are low and to add mass at those sites where the demands are repeatedly high. It is worth emphasizing that, were the removal and deposition of bone tissue to occur independently of mechanical considerations, fluctuations in systemic needs for calcium and magnesium could very well be disastrous for the integrity of the skeleton. Hence, bone is a well-designed organ system whose homeostasis depends on the processing of external mechanical input and physiological signals from the systemic environment and the transduction of these signals into cellular and chemical events.
2.2
Composition and organization of bone
Bone is a composite material consisting of an inorganic and an organic phase. By weight, approximately 60% of the tissue is inorganic matter, 8%–10% is water, and the remainder is organic matter . By volume, these proportions are approximately 40%, 25%, and 35%. The inorganic phase is an impure form of hydroxyapatite (Ca 10 (PO 4 ) 6 (OH) 2 ), which is a naturally occurring calcium phosphate. The organic phase is composed predominantly (98% by weight) of type I collagen and a variety of noncollagenous proteins, and cells make up the remaining 2% of this phase .
2.2.1
Organic phase
The organic phase of bone plays a wide variety of roles, influencing profoundly the structure and also the mechanical and biochemical properties of the tissue. Growth factors and cytokines, and extracellular matrix proteins, such as osteonectin, osteopontin, bone sialoprotein, osteocalcin, proteoglycans, and other phosphoproteins and proteolipids, make small contributions to the overall volume of bone but major contributions to its biologic function (see also Chapter 4 : The skeletal stem cell).
Type I collagen is a ubiquitous protein of extremely low solubility, and it is the major structural component of the bone matrix. The type I collagen molecule consists of three polypeptide chains composed of approximately 1000 amino acids each. These chains take the form of a triple helix of two identical 1(I) chains and one unique 2(I) chain cross-linked by hydrogen bonding between hydroxyproline and other charged residues. This produces a very rigid linear molecule that is approximately 300 nm in length. Each molecule is aligned with the next in a parallel fashion and in a quarter-staggered array to produce a collagen fibril. The collagen fibrils are then grouped in bundles to form the collagen fiber. Within the collagen fibril, gaps known as “hole zones” are present between the ends of the molecules. In addition, pores exist between the sides of parallel molecules ( Fig. 2.1 ). Noncollagenous proteins or mineral deposits can be found within these spaces, and mineralization of the matrix is thought to be initiated in the hole zones.
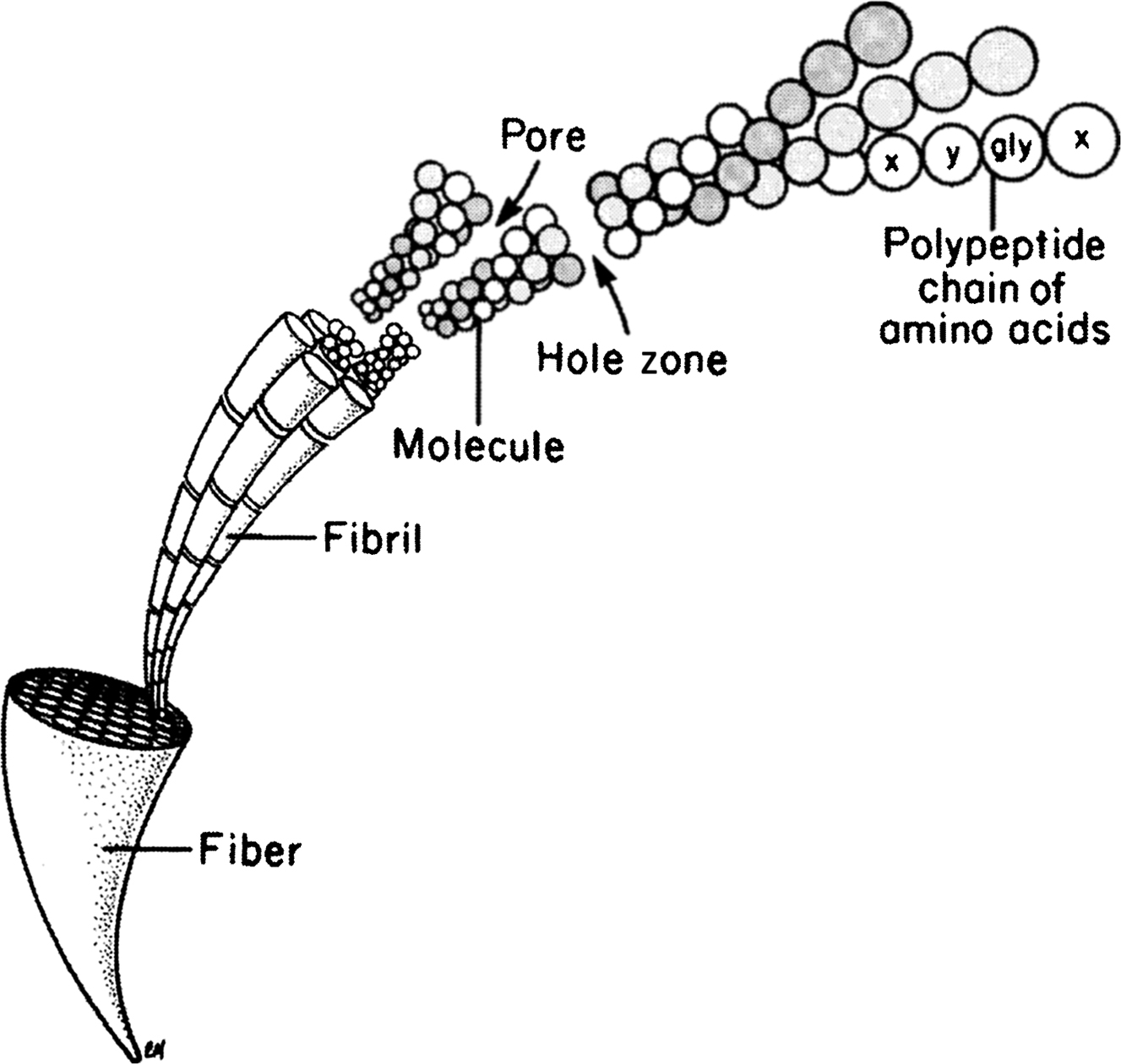
Several noncollagenous proteins have been identified in bone. One of the more extensively studied of these in bone is osteocalcin (OC) or bone-carboxyglutamic acid-containing protein (bone Gla protein). This is a small (5.8 kDa) protein in which three glutamic acid residues are carboxylated as a result of a vitamin K-dependent, posttranslational modification. The carboxylation of these residues confers on this protein calcium and mineral binding properties. Osteocalcin is one of the most abundant noncollagenous proteins in bone, accounting for 10%–20% of the noncollagenous protein content, and it is closely associated with the mineral phase. Evidence suggests that this bone-specific protein may regulate activities of osteoclasts and osteoclast precursors. However, through characterization of the phenotype of osteocalcin-deficient mice, it was also found that osteocalcin has an important role in inhibiting bone formation and in mineral maturation .
Other noncollagenous proteins found in bone may also be important in mineral binding, including nucleation and crystal growth. In addition, several of the bone matrix proteins, such as osteopontin, bone sialoprotein, bone acidic glycoprotein, thrombospondin, and fibronectin, contain arginine–glycine–aspartic acid (RGD) sequences. These amino acid sequences, which are characteristic of cell-binding proteins, are recognized by a family of cell membrane proteins known as integrins. The integrins span the cell membrane and provide a link between the extracellular matrix and the cytoskeleton of the cell. Integrins on osteoblasts, osteoclasts, and fibroblasts provide a means for anchoring these cells to the extracellular matrix. Once anchored, the cells are then enabled to express their phenotype and conduct the type of activities that characterize their functions .
Growth factors and cytokines such as transforming growth factor-β (TGF-β), insulin-like growth factor (IGF), osteoprotegerin (OPG), interferon-γ, the tumor necrosis factors (TNFs), the interleukins, and the bone morphogenetic proteins (BMPs 2–10) are present in very small quantities in bone matrix. Such proteins have important effects regulating bone cell differentiation, activation, growth, and turnover (see Chapter 14 : Cellular and molecular mechanotransduction in bone). It is also likely that these growth factors serve as coupling factors that link the processes of bone formation and bone resorption ( Table 2.1 ).
Structural matrix proteins | |
---|---|
Osteocalcin | Restricted to the osteoblast lineage. Vitamin K dependent. May regulate osteoclasts and their precursors |
Osteopontin | Expressed by a variety of cells. Highly expressed in bone and inflammatory tissue. Contains an RGD sequence. Supports osteoblast attachment to bone. Member of the small integrin-binding ligand N-linked glycoprotein I (sibling) family. Binds and activates MMP-3 |
Bone sialoprotein | Made by osteoblasts and hypertrophic chondrocytes. May initiate mineralization. Supports cell attachment. Binds Ca+ with a high affinity. Member of the sibling family. Binds and activates MMP-2 |
Decorin | Also known as chondroitin sulfate proteoglycan I. Regulates collagen fibrillogenesis and TGFβ1 activity. Binds to fibrinogen |
Biglycan | Also known as chondroitin sulfate proteoglycan II. Involved in the regulation of fibrillogenesis. Modulates BMP 2 induced osteogenesis |
Osteonectin | Expressed in a variety of connective tissues. Strong affinity for Ca+. May play a role in matrix mineralization |
Enzymatic mark modifiers | |
MMPs | The MMPs includes collagenases (MMP-1 and -13) and gelatinases (MMP-2 and -9) |
MMPs are required for collagen degradation. Most are expressed in mature chondrocytes and osteoblasts | |
TIMPS | TIMPs are the inhibitors of MMP activity |
Lysyl oxidase | Copper-dependent extracellular enzyme that catalyzes oxidative deamination of elastin and collagen precursors leading to the formation of a mature ECM |
Stromelysin | Member of the MMP family IMMP-3. Degrades most components of the ECM. Activates other MMPs |
Bone morphogens | |
TGFβ superfamily | The transforming growth factor β (TGFβ) superfamily of morphogens include TGFβ-3, the hone morphogenic proteins BMPs, and the GDFs. This family of morphogens regulates most steps in chondrogenic, osteogenic, and osteoclastogenic cellular differentiation |
FGFs | Fibroblast growth factors I and 2 have angiogenic properties. FGFs promote cellular proliferation |
PDGFs | Platelet-derived growth factors exist in three forms (AA, AB, and BB). PDGF k associate with mesenchymal cell chemotaxis and proliferation |
2.2.2
Inorganic phase
Bone mineral is not pure hydroxyapatite. The small, plate-shaped (20–50 nm long, 15 nm wide, and 2–5 nm thick) apatite crystals contain impurities, most notably carbonate in place of the phosphate groups. The concentration of carbonate (4%–6%) makes bone mineral similar to a carbonate apatite known as dahllite. Other documented substitutions are potassium, magnesium, strontium, and sodium in place of the calcium ions and chloride and fluoride in place of the hydroxyl groups . These impurities reduce the crystallinity of the apatite , and in doing so may alter certain properties such as solubility . The solubility of bone mineral is critical for mineral homeostasis and bone adaptation.
The crystal size and crystallinity of bone mineral are altered with certain diseases and therapies. For example, crystal size is decreased with Paget’s disease and diabetes but increased in osteopetrotic individuals and with bisphosphonate treatment . Whether osteoporosis is associated with abnormal crystal size or crystallinity is the subject of some controversy .
2.2.3
Organization of bone
The skeleton is composed of two parts: the axial skeleton, which includes the bones of the head and trunk, and the appendicular skeleton, which includes all of the bones of the limbs and pelvic girdle. The standard example used in discussions of the macroscale structure of whole bones is the long bone. Long bones such as the tibia, femur, and humerus are divided into three parts: the epiphysis, metaphysis, and diaphysis ( Fig. 2.2 ). The epiphysis is found at either end of the bone and develops from a center of ossification that is distinct from the rest of the long bone shaft. It is separated from the rest of the bone by a layer of growth cartilage known as the physis . The metaphysis is the region between the physis and the central portion of the long bone (known as the diaphysis). From a structural perspective the metaphysis is the region of transition from the wider epiphysis to the more slender diaphysis.
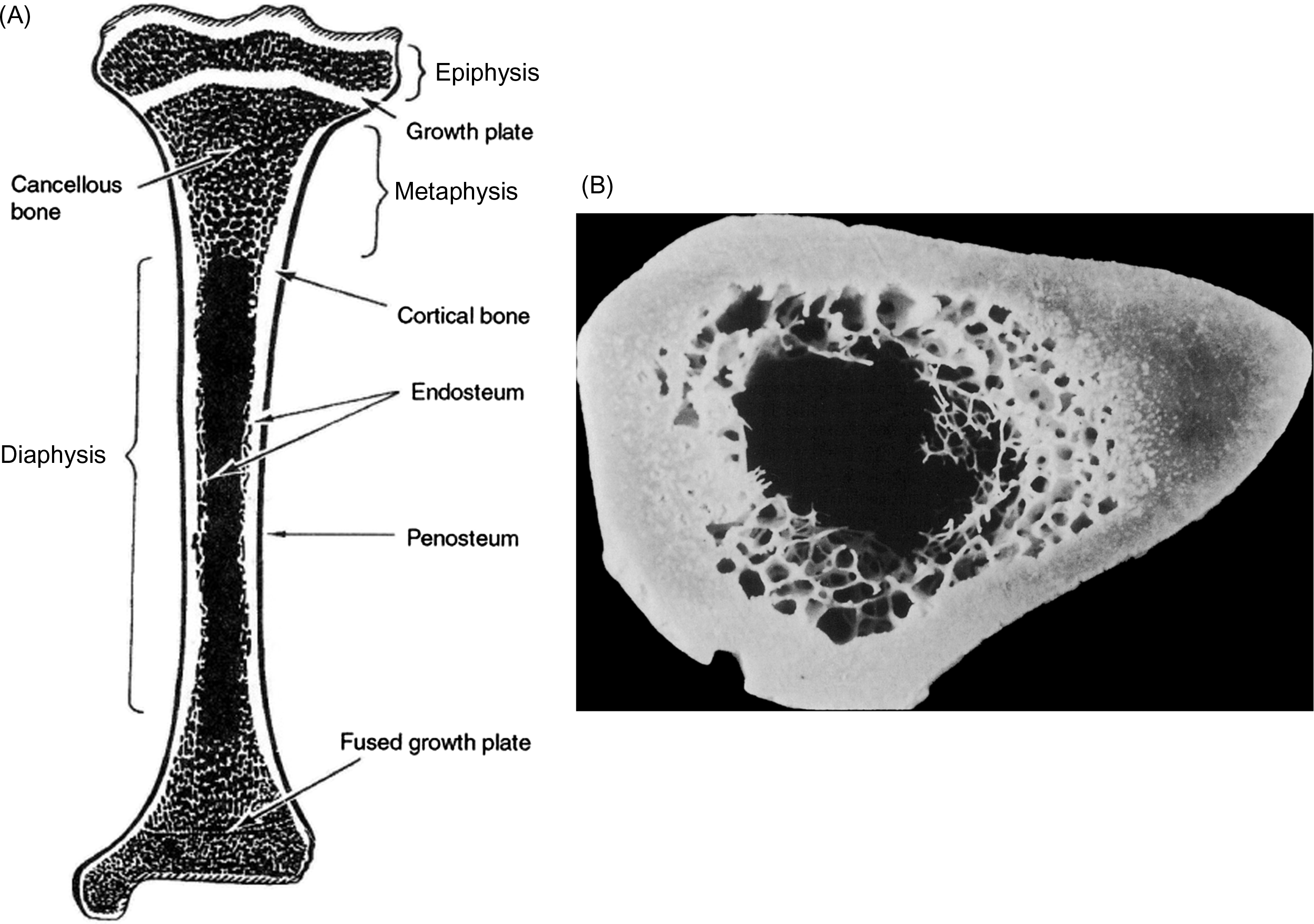
Membranes on both the outer and inner surface of the whole bone play important roles in bone modeling and remodeling, as well as in fracture healing. The periosteum lines the outer surface of nearly the entire long bone ( Fig. 2.2 ). It is not present on the articulating surfaces and at ligament and tendon insertion points. The periosteum is composed of two layers: an outer fibrous layer, that is in direct contact with muscle and other soft tissues, and an inner layer, known as the cambium layer. Although the outer layer is much like a sheath of fibrous connective tissue, the cambium layer is populated by uncommitted progenitors of osteoblasts and chondrocytes ( Fig. 2.3 ). Through this pool of precursor cells the periosteum contributes to appositional bone growth during bone development and is responsible for the expansion of the diameters of the long bones with aging. The endosteum lines the inner surfaces of the long bone and consists of bone surface cells, including osteoblasts and bone lining cells ( Fig. 2.2 ).
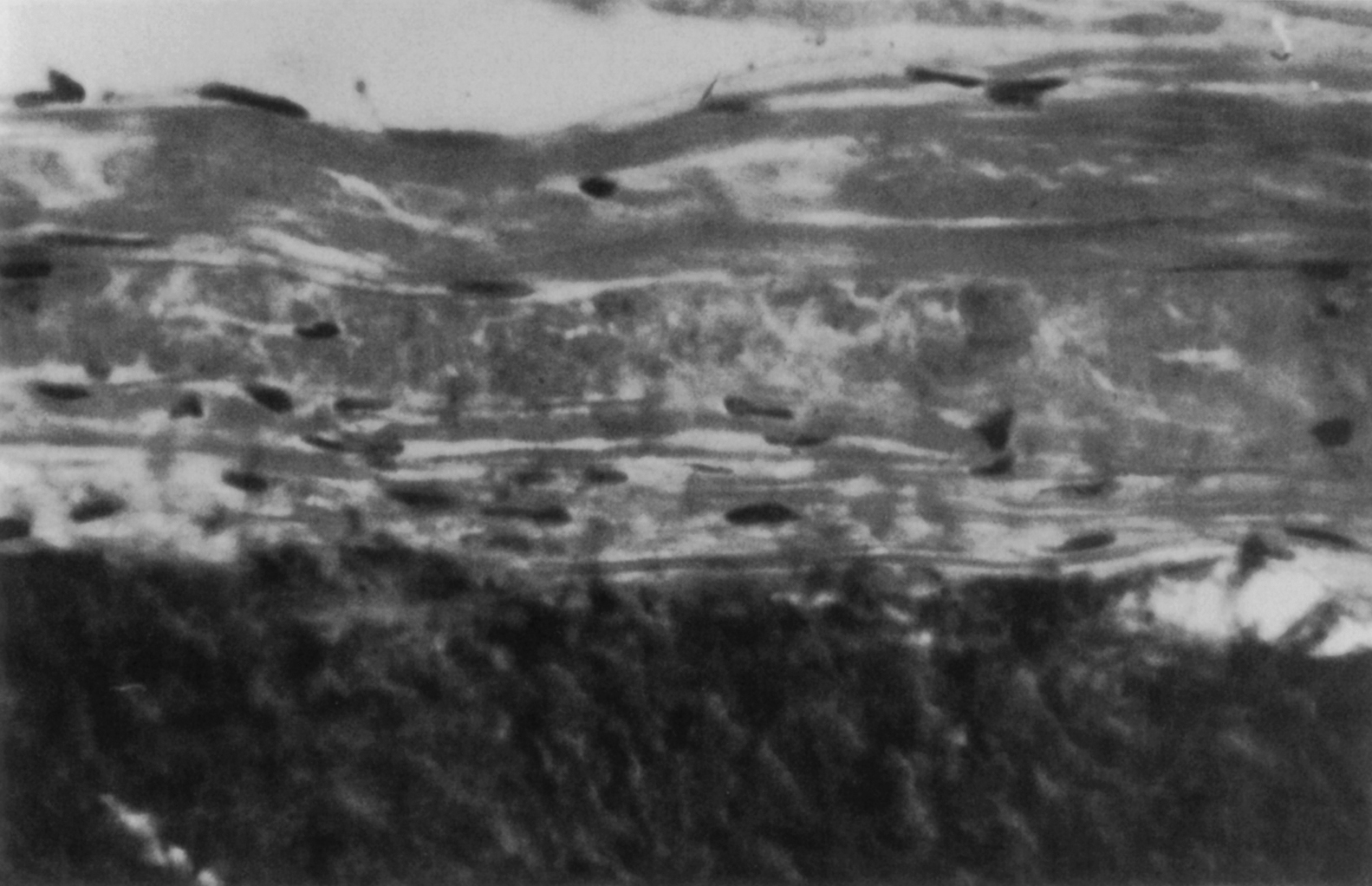
The building block of bone tissue is the mineralized collagen fibril (approximately 0.1–3 µm in diameter). These fibrils are arranged either as a collection of randomly oriented fibrils known as woven bone ( Fig. 2.4 ) or as aligned in thin sheets called lamellae which are then stacked in a plywood type arrangement known as lamellar bone ( Fig. 2.5 ). Woven bone is considered immature or primitive bone and is normally found in the embryonic and newborn skeletons, in fracture callus, and in some metaphyseal regions of the growing skeleton. Given that fracture healing and skeletal growth are scenarios in which rapid deposition of bone tissue is advantageous, it is perhaps not surprising that woven bone is laid down relatively quickly (as much as 4 µm/day compared to 1 µm/day for lamellar bone). Woven bone is also found in certain bone tumors, in patients with osteogenesis imperfecta, and in patients with Paget’s disease. Lamellar bone is the more mature form of bone tissue that results from the remodeling of woven bone or preexisting lamellar tissue. Lamellar bone begins to develop in the human skeleton at approximately 1 month of age, and by the age of 4, most of the bone in the body is lamellar.
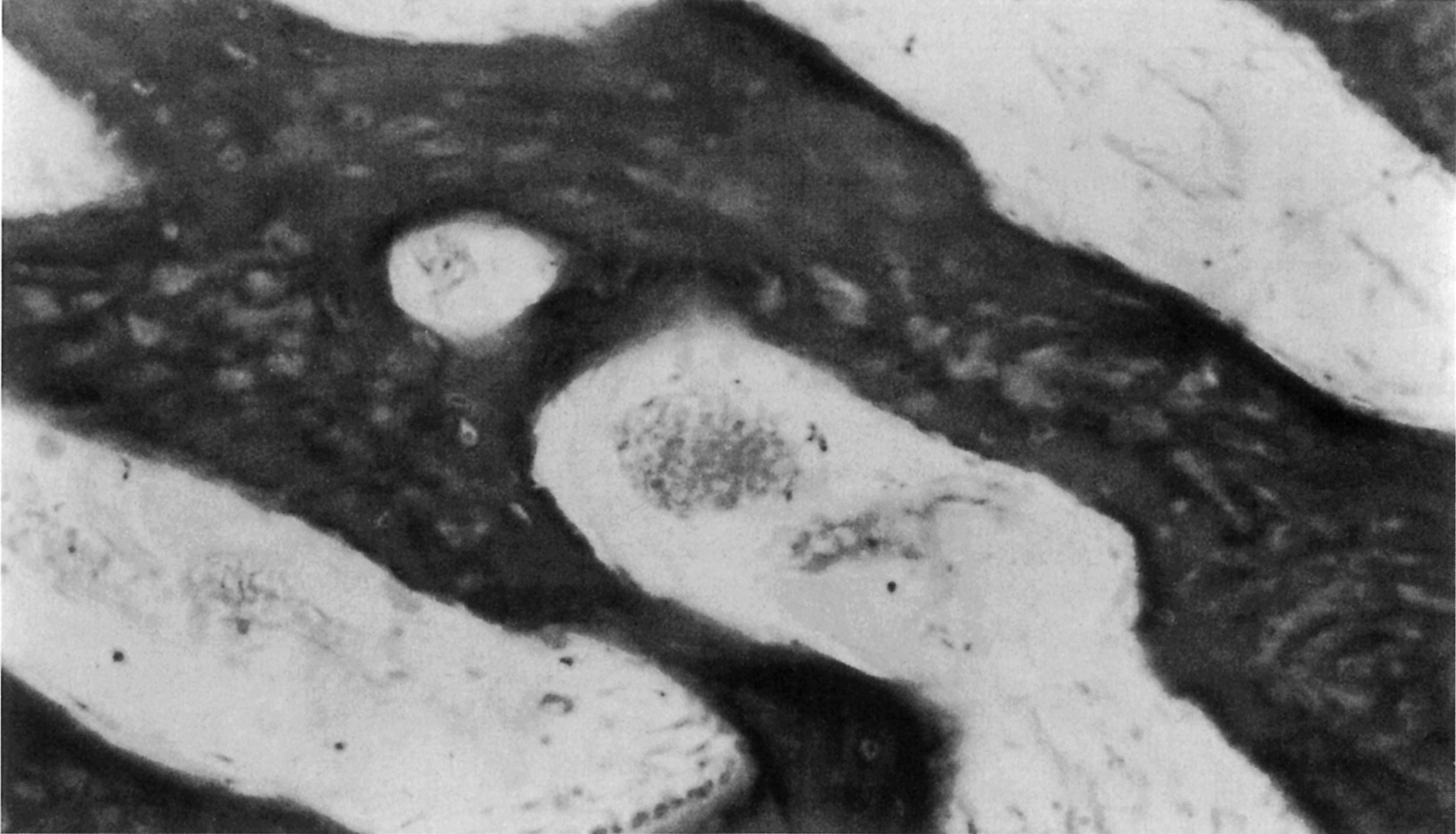
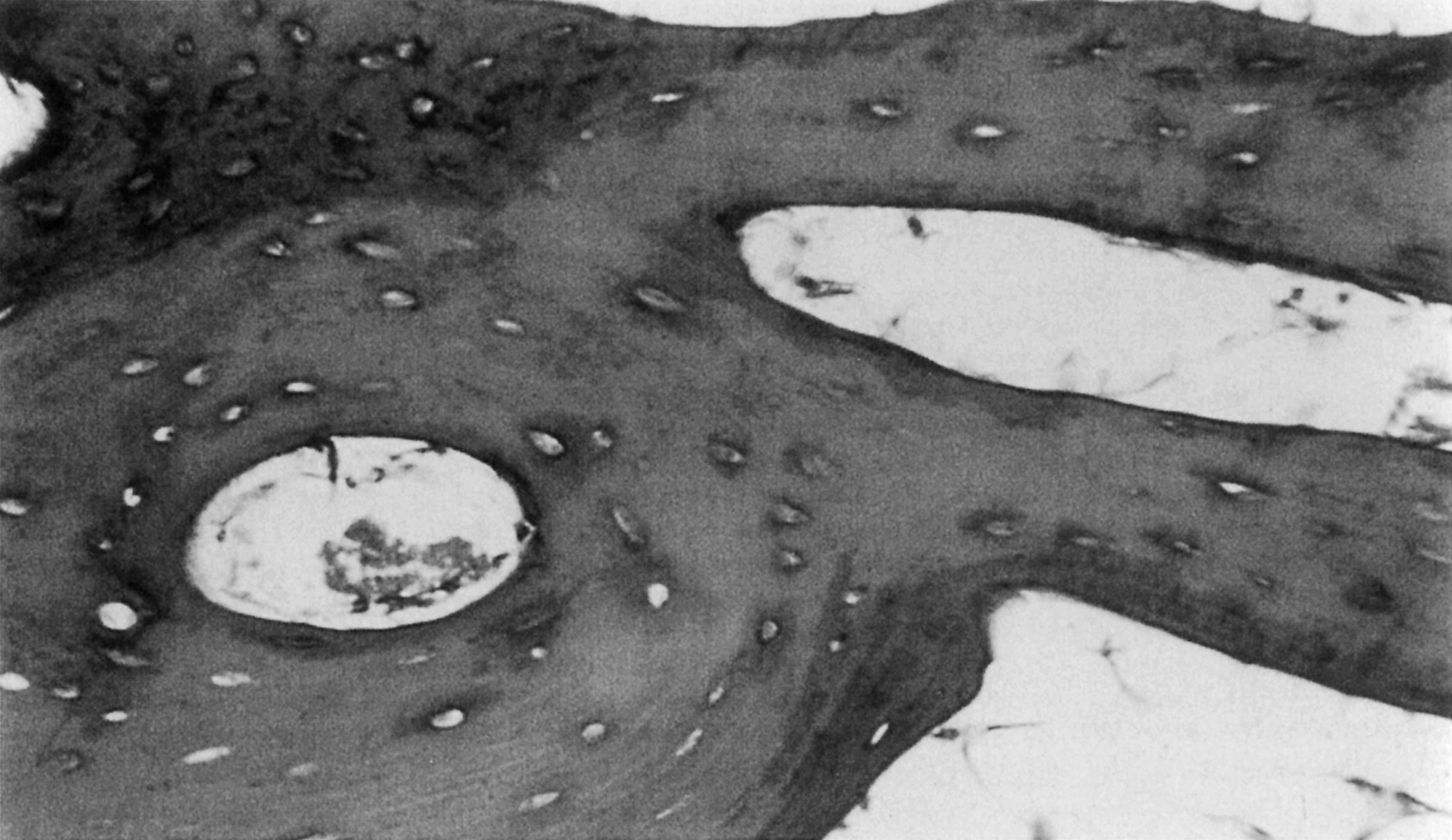
In addition to the difference in fibril arrangement, woven and lamellar bone differ somewhat in composition. As compared to lamellar bone, woven bone has a smaller average apatite crystal size and higher cell density, and the distribution of osteocytes appears random rather than closely associated with the mineralized fibril structure ( Figs. 2.4 and 2.5 ). Newly formed woven bone is not as highly mineralized as lamellar bone, although the opposite is true when comparing the final degree of mineralization in these two types of tissue. The differences in composition and structure lead to differences in the mechanical behavior. Due to the random orientation of the fibrils, woven bone is more isotropic than lamellar bone, that is, its mechanical properties such as stiffness and strength do not depend on the direction in which the forces are applied. In contrast the stiffness and strength of individual lamellae are greatest in the direction of the fibrils. Depending on the distribution of fibril orientation throughout a region of lamellar bone, however, the stiffness and strength of lamellar bone can range from anisotropic (direction-dependent) to nearly isotropic.
In both woven and lamellar bone the osteocytes reside in small ellipsoidal holes (5 µm minor diameter; 7–8 µm major diameter) called lacunae ( Fig. 2.6 ). In lamellar bone the lacunae are located along the interfaces between lamellae. There are about 25,000 lacunae per mm 3 in bone tissue; this number and also the size of the lacunae decrease with age , although it is not clear if these characteristics are further altered with diseases such as osteoporosis . Each osteocyte has dendritic processes that extend from the cell through tiny (≈0.5 µm diameter, 3–7 µm long) channels called canaliculi, to meet at cellular gap junctions with the processes of surrounding cells. There are about 50–100 canaliculi per single lacuna and about 1 million/mm 3 of bone tissue. The lacunar–canalicular network may play a central role in bone mechanotransduction and can be profoundly disrupted through aberrations in perilacunar remodeling (osteocytic osteolysis) .
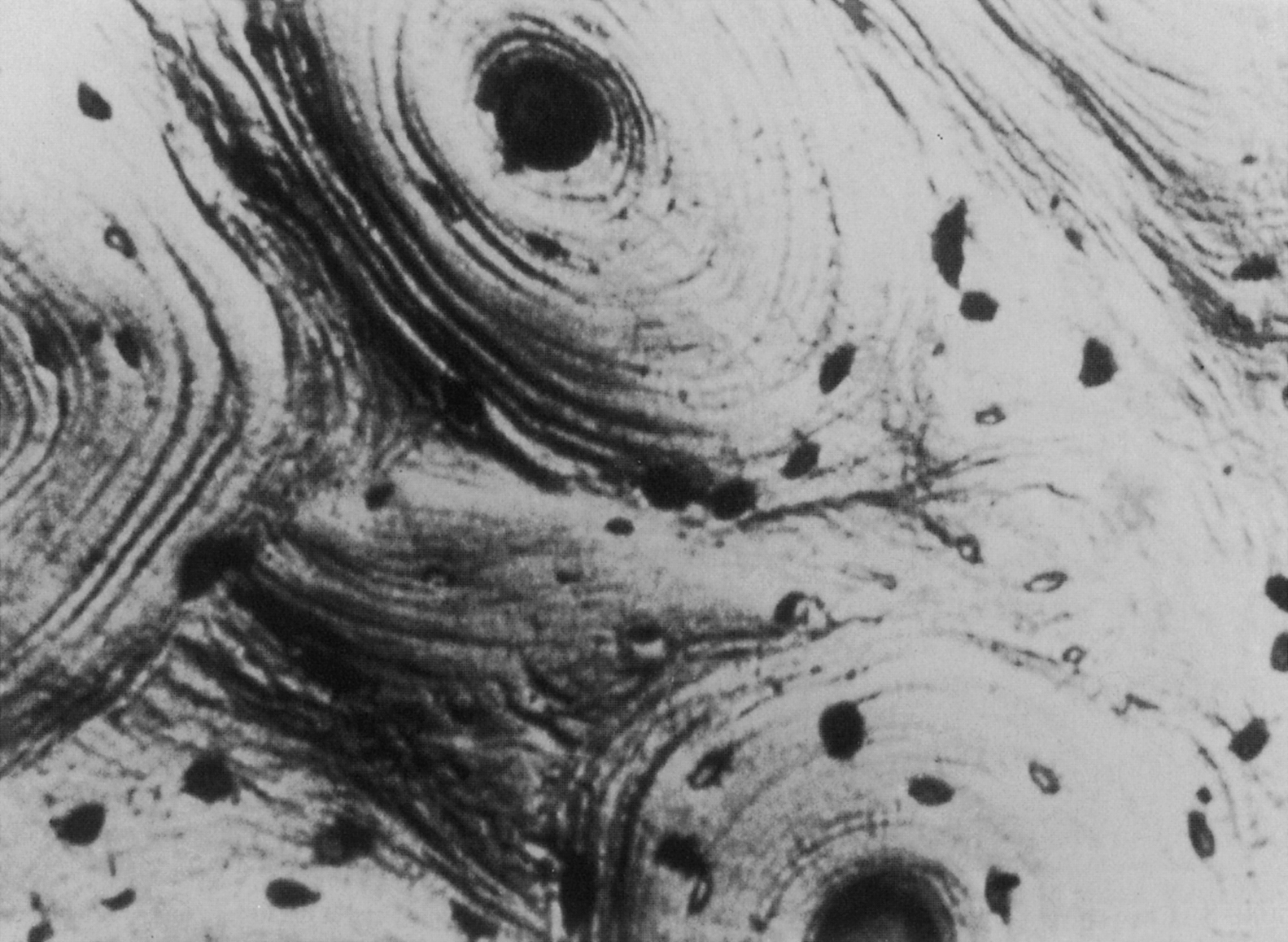
Both woven and lamellar bone can occupy fairly large volumes, extending uniformly throughout volumes as large as several cubic millimeters. In particular, lamellar bone is found in the long bone diaphysis as large concentric rings of lamellae in the outer 2–3 mm of the circumference. However, lamellar bone is also commonly arranged in smaller cylindrical structures called secondary osteons or Haversian systems. These osteons are termed “secondary,” because they are formed through bone remodeling, replacing the previous, “primary” bone tissue. Their diameter and length (typically 200 µm and 1–3 mm, respectively) are determined by the diameter and length of the cutting cone, which is described in the next section on bone remodeling. Each osteon consists of 10–30 concentric rings of lamellae that surround a central cavity, the Haversian canal , containing one or more blood vessels and nerves ( Fig. 2.6 ). A second type of canal, the Volkmann’s canals, run transverse to the osteonal axis, providing a radial path for blood flow through the whole bone. The outer surface of the osteon is lined with a thin (1–2 µm) layer, known as the cement line, consisting of calcified mucopolysaccharides and very little collagen . In the diaphysis, secondary osteons are typically oriented such that their longitudinal axis is aligned with the diaphyseal axis; although evidence exists that in some bones, the osteons loosely spiral around the diaphyseal axis . Although these osteons are often viewed in cross section, it is important to note that in three dimensions, the osteon is an irregular, anastomosing cylinder.
Most vessels in Haversian and Volkmann’s canals have the ultrastructural features of capillaries, although some smaller sized vessels may resemble lymphatic vessels. When examined histologically, these small vessels contain only precipitated protein; their endothelial walls are not surrounded by a basement membrane. The basement membrane of capillary walls may function as a rate-limiting or selective ion-limiting transport barrier, because all material traversing the vessel wall must go through the basement membrane. The presence of this barrier is particularly important in calcium and phosphorus ion transport to and from bone. The capillaries in the central canals are derived from the principal nutrient arteries of the bone: the epiphyseal and metaphyseal arteries. The vascular system is critical for bone function, not only with respect to nutrient supply but also as a source of cells of both the osteoclast and osteoblast lineage .
At the scale of 1–10 mm, there are two types of bone: trabecular bone (also known as cancellous or spongy bone) and cortical bone (also known as compact or dense bone). Trabecular bone is found principally in the axial skeleton and in the metaphyses and epiphyses of long bones ( Fig. 2.2 ). It is a highly porous structure consisting of a network of rod- and plate-shaped trabeculae surrounding an interconnected pore space that is filled with bone marrow ( Fig. 2.7 ). Trabeculae range in thickness from 50 to 300 µm and are comprised, almost exclusively, of lamellar bone arranged in packets that are sometimes referred to as hemiosteons. However, the thicker trabeculae can contain secondary osteons, presumably because their thickness is such that nutrient transport via the lacunar–canalicular network alone is insufficient. In the mature human skeleton, cortical bone consists largely of secondary osteons and, to a lesser extent, circumferential lamellae that ring the outer surface of the diaphysis and a type of lamellar bone known as interstitial bone ( Fig. 2.8 ). Interstitial bone is merely composed of portions of secondary osteons that were not removed by a cutting cone during remodeling. Both the metaphyses and epiphyses of long bones have a thin shell of cortical bone surrounding the trabecular compartment, and the diaphysis is entirely cortical ( Fig. 2.2 ).
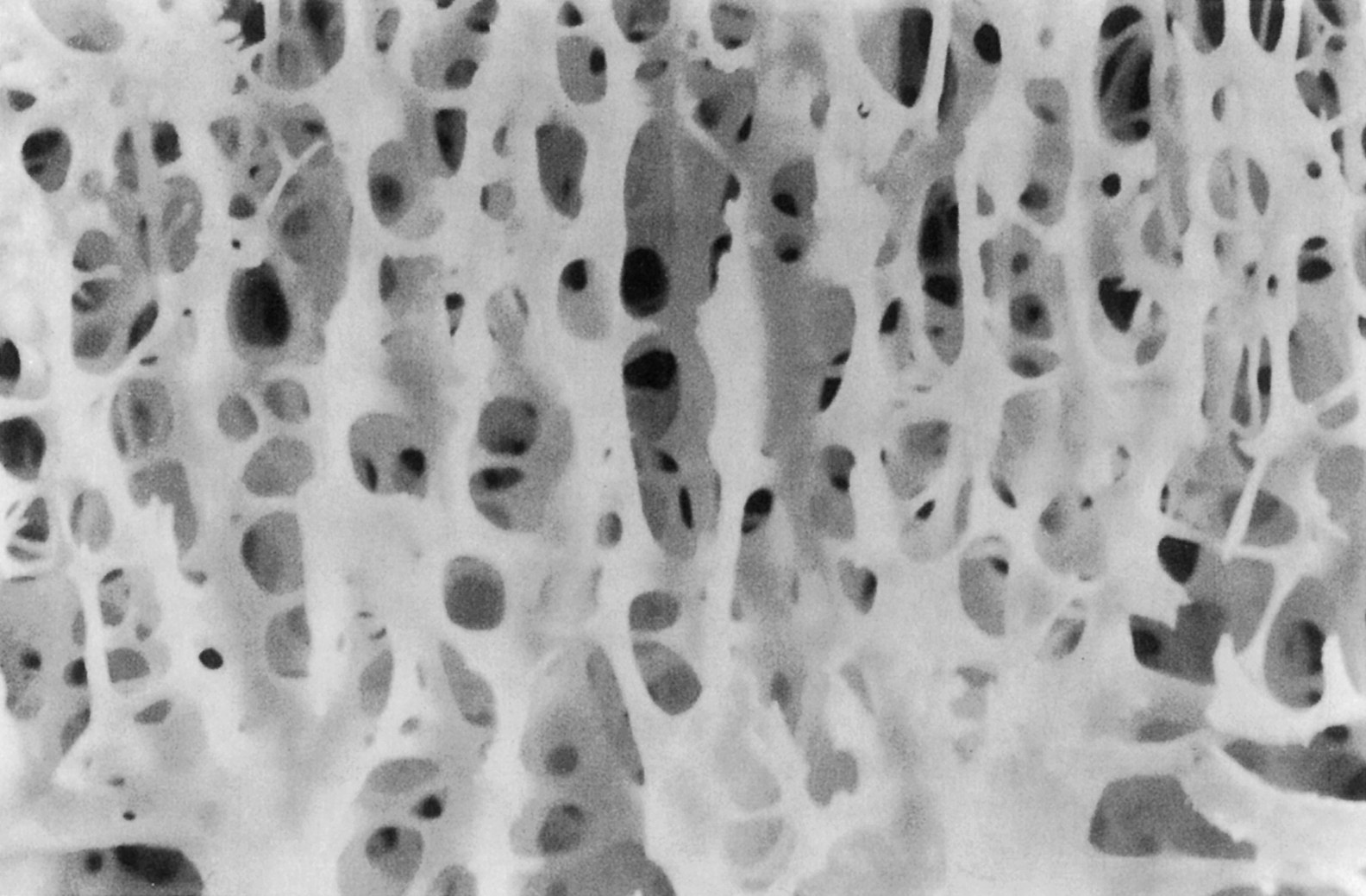
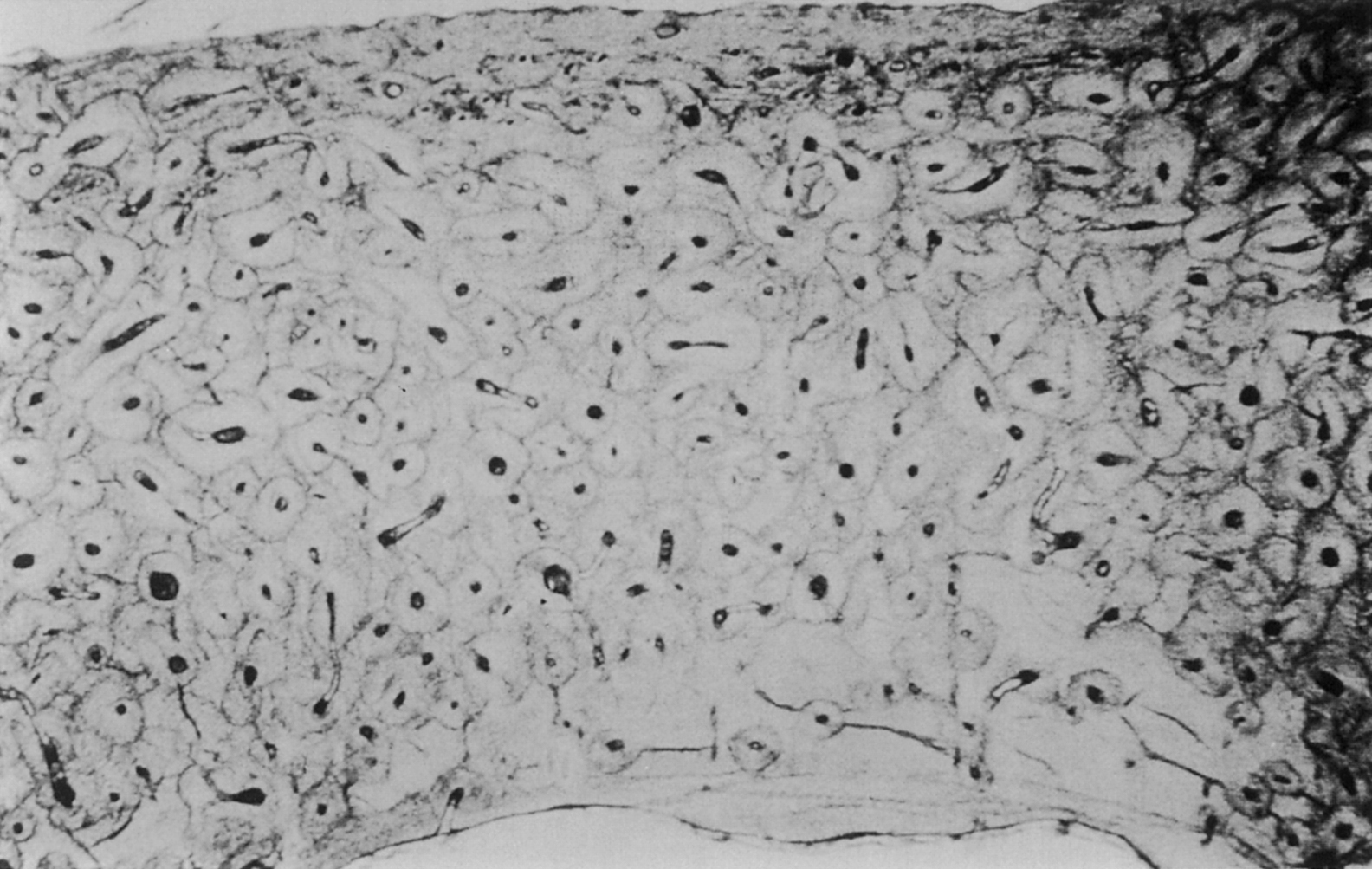
The distinction between cortical and trabecular bone can be made largely on the basis of porosity. The porosity of cortical bone ranges only 5%–20% and is due to the Haversian and Volkmann’s canals and, to a lesser extent, the lacunar and canalicular spaces. Trabecular bone has another scale of porosity due to the marrow space; typical spacing between trabeculae ranges 100–500 µm. The porosity of trabecular bone can range from 40% in the primary compressive group of the femoral neck to more than 95% in the elderly spine. Porosity is the major determinant of the stiffness and strength of trabecular bone .
In addition to porosity the three-dimensional structure of trabecular bone, known as the trabecular architecture , can vary tremendously among anatomic sites and with age. Trabecular bone from the vertebral body tends to be predominantly rod-like, while that from the proximal femur contains a more balanced mixture of rods and plates ( Fig. 2.9 ). Quantitative descriptors of trabecular architecture such as trabecular thickness and trabecular spacing contribute somewhat independently of porosity to trabecular bone stiffness and strength .
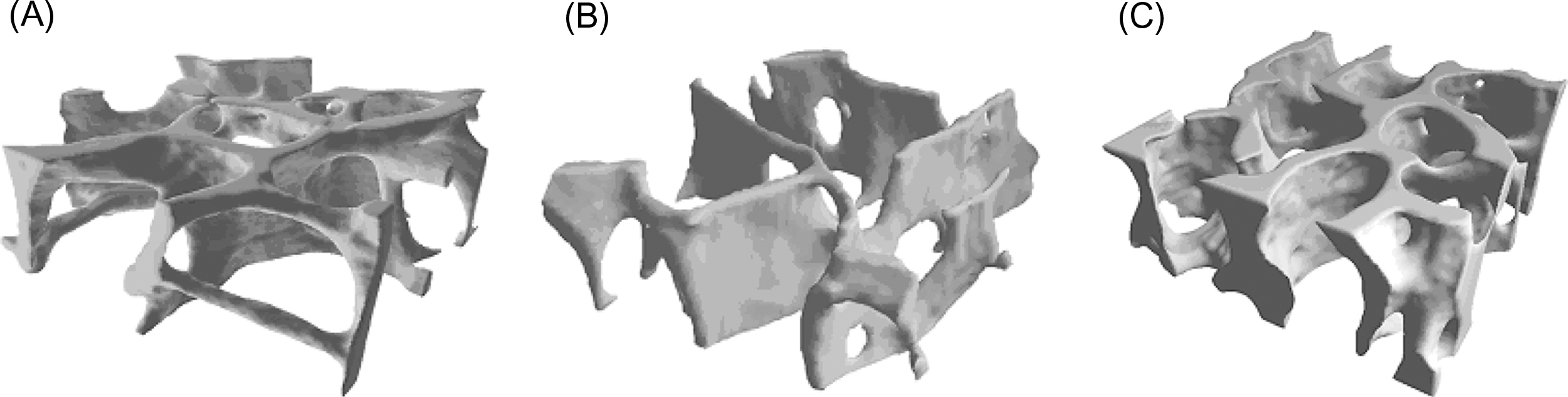
With age and also with disuse, trabeculae become progressively thinner and can become perforated by resorption cavities. In certain anatomic sites such as the vertebral body and proximal tibia, age-related changes in trabecular architecture include an increase in the anisotropy of the trabecular structure ( Fig. 2.10 ) . With the overall decrease in bone mass with age, this increase in anisotropy helps to preserve the load-carrying capacity of trabecular bone along its main “grain” axis, but at the necessary expense of the load-carrying capacity in other directions. Nonhabitual loading conditions such as impact after a fall can subject trabecular bone to such off-axis loads. Thus the risk of fracture due to off-axis loads can increase with age to a greater extent than the decrease in bone mass alone would suggest.
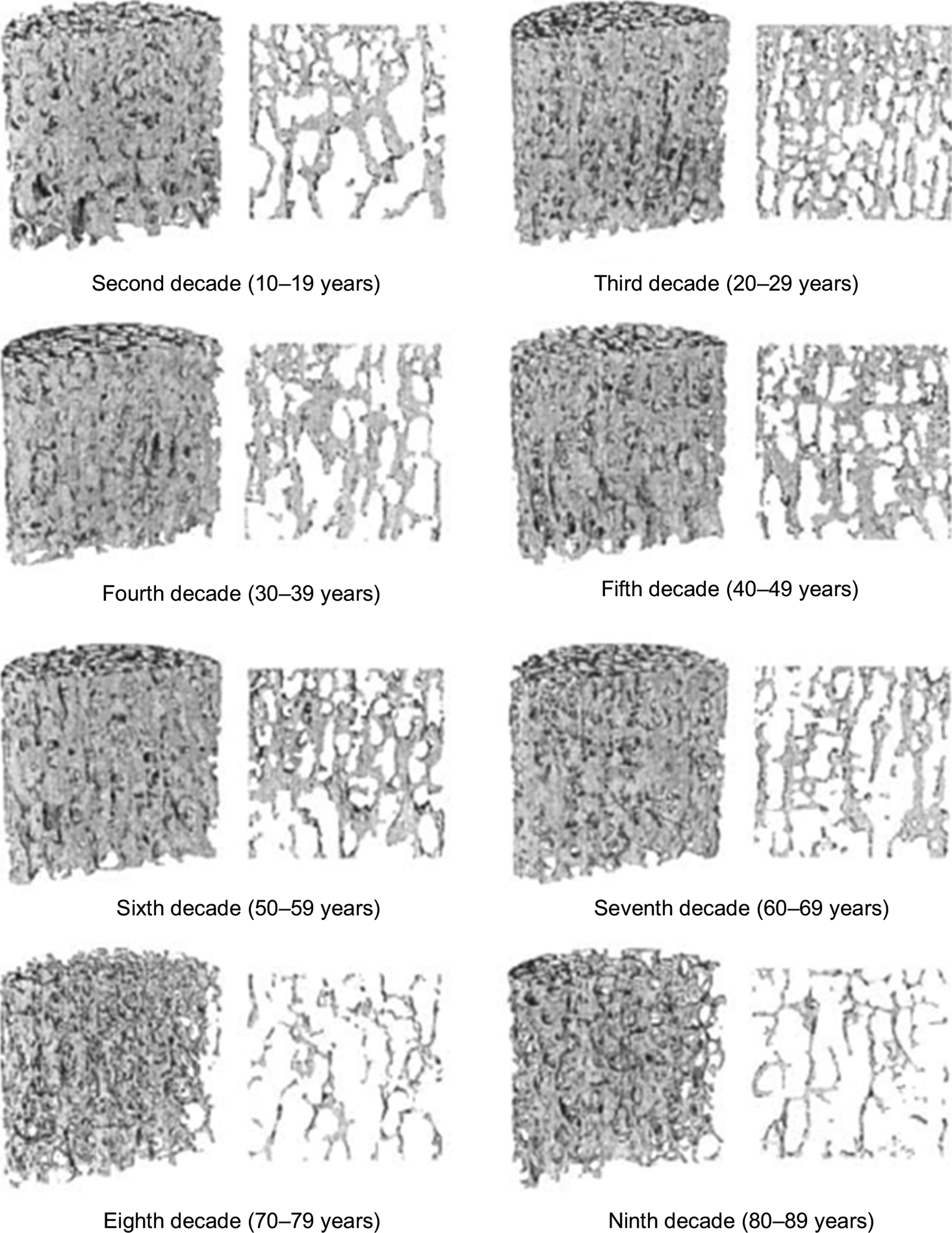
2.3
Cellular components of bone
2.3.1
Bone cells
Bone metabolism is regulated by multiple environmental signals, including chemical, mechanical, electrical, and magnetic. The local cellular compartment of the bone responds to these environmental signals by modulating the balance between new bone formation and the local resorption of older bone (i.e., remodeling). Three cell types are typically associated with bone homeostasis: osteoblasts, osteocytes, and osteoclasts. These three cell types are derived from two separate stem cell lineages, the mesenchymal lineage and the hematopoietic lineage, underscoring the unique regulation of bone homeostasis and the intimate interactions between the immune system and bone.
2.3.2
Mesenchymal lineage cells
Bone formation, both embryonic and postnatal, is carried out by the mesenchymal lineage osteoblast. As noted previously, osteoblasts produce the protein matrix of bone made up of osteoid, creates a template for mineralization and production of the mature bone. In addition to bone formation, osteoblasts assist with the initiation of bone resorption by secreting factors that recruit and promote the differentiation of monocytic lineage cells into mature osteoclasts and also by producing neutral proteases that degrade the osteoid and prepare the bone surface for osteoclast-mediated remodeling.
The stem cells giving rise to osteogenic cells were initially described as arising from a multipotent stem cells named the mesenchymal stem cells (MSCs). This initial designation was defined empirically based on finding both from studies that were carried out in vitro on dissociated cells from embryonic limb bud cells and several stably transformed mouse lines showing that under selective in vitro growth conditions, MSCs can differentiate into a variety of cell types, including myoblasts, adipocytes, chondrocytes, osteoblasts, and osteocytes . An aspect of the concept of MSCs was that they were distributed throughout vertebrates in most tissues of mesodermal origin. This understanding was based on a large number of studies assessing a variety of cell populations derived from the bone marrow , various peripheral fat depots , muscle , abluminal surface of vessels , and periosteum , which showed that when cells were grown in vitro under appropriate conditions, they would give rise to osteogenic, chondrogenic, and adipogenic cell types. As interest in the therapeutic potential of MSCs expanded, fluorescense-activated cell sorting (FACS) analysis was applied as it had been used in hematopoietic field, in an attempt to define common features of MSCs. This led the International Society of Cellular Therapy to develop a consensus minimal criteria for multipotent MSCs. They suggested that cells must be adherent to plastic; show multipotent differentiation in vitro to osteoblasts, adipocytes, chondrocytes; and be CD105, CD73, and CD90 positive and CD45, CD35, CD14, CD79 alpha, and HLA-DR negative .
Over the last several years, several concepts concerning MSCs have been under revision. With the advent of the use of transgenic lineage tracking studies and further assessments using flow activated cell sorting, the concept that there is a single MSC type that is broadly distributed throughout mesodermal-derived tissues has been challenged . Data from several studies now suggest that cells that contribute to skeletal tissue homeostatic maintenance and to injury repair are more restricted to within the skeletal organs and have been renamed skeletogenic stem cell (SSC). Several recent studies suggest that there may be multiple SSC populations that are specified by the compartment of bone in which they reside (periosteal vs marrow) and which change or become more restricted with aging . Several studies also show that specific stem cell populations and even more committed cells exhibit plasticity under given developmental conditions or in response to injury. Finally, a number of studies suggest that a large number of cells that contribute to bone injury repair and homeostatic maintenance of bone are not actually stem cells but are largely committed osteogenic progenitors .
The specific morphogenetic factors and transcriptional determinants that promote skeletogenic lineage selection of various stem cell populations involve a number of coordinated lineage selection steps whose activities are modulated in response to the local micro-environment ( Fig. 2.11 ). The first of these steps in the development of the axial and appendicular skeleton is a commitment step to a common osteo/chondral progenitor though the activation the activities of Sox 9 . Subsequent to this initial step, two transcription factors have been demonstrated to be required for osteoblast formation and differentiation, Runx2 and Osterix . The regulatory activity of these central osteoblast regulators is modified by cofactors including the members of the Dlx (distaless), Msx, and Hox homeodomain gene families and downstream signal transduction mediators such as the TGFβ superfamily-related SMADs. Runx2 is a member of the runt homology domain transcription factors and acts as a scaffolding protein organizing nuclear complexes at discreet sites on the nuclear matrix associated with active gene transcription. Transgenic knock-out studies have clearly demonstrated the requirement for Runx2 activity for osteoblast differentiation, as Runx2 knock-out mice produce no bone during embryogenesis . Runx2 knock-out mice lack osteoblasts and display defects in chondrocyte hypertrophy demonstrating the role of Runx2 in both osteoblast differentiation and chondrocyte maturation. Runx2 regulates the expression of many mature osteoblast-related genes, including osteocalcin, bone sialoprotein, osteopontin, and collagen type I. The second required transcription factor for osteoblast differentiation is the zinc finger motif containing factor Osterix. Like Runx2 knock-out mice, Osterix knock-outs lack embryonic bone formation and osteoblast differentiation . Unlike Runx2 deficient animals, Osterix knock-outs do not display the defects in chondrocyte hypertrophy, and Runx2 expression levels are comparable to controls. Osterix functions downstream of Runx2 activity as Runx2 −/− cells express no Osterix. While the mechanism through which Osterix regulates osteoblast differentiation is poorly understood, it has been noted that in Osterix knock-out mice, the pool of Runx2-expressing preosteoblasts express several genes associated with chondrogenesis suggesting Osterix plays a role in stabilizing osteogenic commitment and osteoblast maturation.
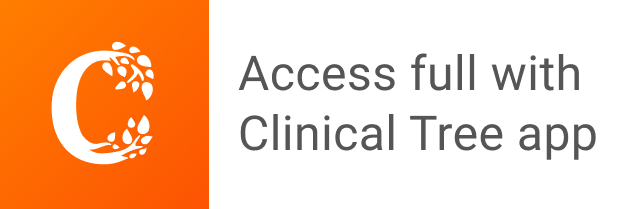