Telomeres, Telomerase, and Cancer
Kwok-Kin Wong
Norman E. Sharpless
Ronald A. Depinho
Maintenance of most adult organ systems requires extensive cell renewal, typified most strikingly by the replacement of the intestinal lining on a weekly basis and the production of trillions of new blood cells daily. Yet a lifetime of factors including continual telomere erosion, errors in DNA replication, intrinsic and carcinogeninduced somatic mutations, cancer-relevant germline variants, and epigenetic insults conspire to endow cells with the large number of changes needed for malignant transformation. How is it that replicating tissues, showered with myriad cancer-relevant somatic alterations, resist malignant transformation? That these mutations are indeed present in normal human tissues is reflected by the remarkable observations that roughly 1% of neonatal cord blood collections contain significant numbers of myeloid clones harboring oncogenic fusions such as the AML1-ETO fusion associated with acute leukemia,1 and that approximately one-third of adults possess the IgH-BCL2 translocation associated with follicular lymphomagenesis.2 As the prevalence of these cancers is far lower in the general population, these observations imply that potent tumor suppressor mechanisms must be operating to constrain the growth and survival of these aspiring cancer cells.
The most prominent biologic manifestations of an activated tumor suppressor response are apoptosis (cell death) and senescence (permanent cell cycle arrest). These biologic processes are linked to powerful checkpoint effector molecules involving the p16INK4a-Rb pathway, the ARF-p53 pathway, and specialized chromosomal DNA ends termed telomeres. These genetic elements comprise powerful tumor suppressor barriers and act cooperatively to eliminate or to place a limit on the replicative lifespan of rogue would-be cells. The importance of apoptosis in preventing cancer is further discussed in Chapter 7. The focus of this chapter will be on the role of telomere dynamics and associated telomere-related cellular checkpoint processes, particularly senescence, in the regulation of neoplastic transformation. A significant body of clinical and translational science now supports such a role for telomeres and cellular senescence, and in this chapter, we present rapidly increasing clinical data pointing to the relevance of these processes in human disease, particularly cancer. Indeed, it is worth noting that the Nobel Prize for physiology or medicine in 2009 was awarded to Blackburn, Greider, and Szostak for their pioneering and seminal work in telomere biology that advanced our present understanding of aging and cancer.
TELOMERES AND TELOMERASE
Telomere dysfunction is a principal tumor suppressor mechanism manifesting most prominently as apoptosis and senescence. At the same time, when accompanied by functional p53 loss, the genome-destabilizing impact of telomere dysfunction can cause widespread mutations that propel normal cells toward malignant transformation. Thus, the telomere-based anticancer mechanism can actually fuel tumorigenesis in certain contexts. The knowledge of the basic biology of telomeres and telomerase has yielded fundamental insights into both cancer prevention and cancer promotion. The powerful and complex impact of telomere dynamics in model organisms and humans reflects the crucial role of telomere function in processes of genomic instability, organ homeostasis, chronic diseases, aging, and tumorigenesis. With respect to tumorigenesis, the study of telomeres in the mouse has provided insight into how advancing age in humans fuels the development of epithelial cancers as well as how chronic inflammation and degeneration may engender increased cancer risk in affected organs. These advances in the basic understanding of telomere maintenance are now being translated into clinically relevant applications that may have an impact on the diagnosis and management of a broad spectrum of cancers as well as aging, age-related disorders, and degenerative conditions. The important role of telomere biology in aging and degenerative diseases has been reviewed elsewhere.3,4
Telomeres
Telomeres are specialized nucleoprotein complexes at the ends of linear chromosomes consisting of long arrays of double-stranded TTAGGG repeats, a G-rich 3′ single-strand overhang, and associated telomeric repeat binding5,6,7 (Fig. 4.1). The work of Muller and McClintock in the 1930s led to the concept that telomeres function to “cap” chromosomal termini and prevent end-to-end recombination, thereby maintaining chromosomal integrity. Subsequent work has substantiated this model across the animal and plant kingdom, underscoring the critical roles served by the telomere complex.
Telomere structure and function have been studied extensively in mammals. Although the overall structural features of telomeres are preserved among different mammalian organisms, lengths can vary considerably from species to species: for example, 5 to 15 kb for humans versus 20 to 80 kb for the laboratory mouse. On the structural level, electron microscopy and other studies have shown that telomeres form complex secondary and tertiary structures via DNA-DNA interactions between the telomeric repeats, DNA-protein interactions between the telomeric DNA and the telomeric repeat binding proteins (shelterins or telosomes8,9), and protein-protein interactions between the telomeric repeat binding proteins themselves and other associated proteins (Fig. 4.1). The formation of this well-documented, higher-order DNA-protein complex has provided a working model of how the telomere functions as a capping structure, preventing the ends of linear chromosomal DNA from being recognized as either a DNA double-strand break (DSB) or DNA single-strand break, thereby avoiding activation of the DNA damage response and the formation of chromosomal end-to-end fusions through the DNA repair machinery.7
Many proteins involved in DNA DSB repair, including nonhomologous end-joining and homologous recombination processes, have been found to be physically associated with the telomeres.7,8,9,10 These findings have fueled speculation that DSB repair proteins provide a protective role at the telomere; for example, by sequestering the telomere end from the DNA damage surveillance/repair machinery. Experimental support for
this hypothesis has emerged from the mouse, in which germ line inactivation of various repair proteins (e.g., Ku and DNA-PK) results in reduced telomere length or loss of capping function, or both, leading to increased end-to-end fusions.11 Correspondingly, in cultured human cells, experimental disruption of telomere-binding proteins results in the unraveling of higher-order nucleoprotein structure and telomere localization of DNA DSB surveillance/repair proteins (e.g., 53BP1, gamma-H2AX, Rad17, ATM, and Mre11), establishing that dysfunctional telomeres can indeed serve as substrates for the classic DNA repair machinery.12 Recently, elegant in vitro and mouse genetic experiments have shown that subunits of the shelterin complex actively repress the ATM and ATR DNA damage signaling pathways.7,13
this hypothesis has emerged from the mouse, in which germ line inactivation of various repair proteins (e.g., Ku and DNA-PK) results in reduced telomere length or loss of capping function, or both, leading to increased end-to-end fusions.11 Correspondingly, in cultured human cells, experimental disruption of telomere-binding proteins results in the unraveling of higher-order nucleoprotein structure and telomere localization of DNA DSB surveillance/repair proteins (e.g., 53BP1, gamma-H2AX, Rad17, ATM, and Mre11), establishing that dysfunctional telomeres can indeed serve as substrates for the classic DNA repair machinery.12 Recently, elegant in vitro and mouse genetic experiments have shown that subunits of the shelterin complex actively repress the ATM and ATR DNA damage signaling pathways.7,13
A further understanding of the molecular mechanisms governing the repression versus activation of the DNA DSB surveillance/repair apparatus at the telomere could lead to the development of novel cancer therapeutic options. For example, the design of agents that can uncap telomeres while preserving the DNA damage checkpoint response yet neutralize the actual DNA damage repair process would be ideal because they would produce unrepaired DSBs and elicit cell-cycle arrest or apoptosis responses. Lastly, in the near future, agents designed to uncap the telomeres could be used in combination with conventional chemotherapeutic agents that create DSB for cancer treatment, thereby simultaneously targeting these intertwined pathways.
Telomerase
Conventional DNA polymerases operating in the S phase of the cell cycle require an RNA primer for reverse-strand synthesis, resulting in incomplete DNA replication of telomeres during each cell division. The solution to this “end-replication problem” is the telomere-synthesizing telomerase enzyme, a specialized ribonucleoprotein complex with reverse transcriptase activity. The functional telomerase holoenzyme is a large multisubunit complex that includes an essential telomerase RNA (hTERC) component serving as a template for the addition of telomere repeats and a telomerase reverse transcriptase (hTERT) catalytic subunit.14 In some normal human somatic cells, telomerase levels are insufficient to maintain telomere length, resulting in progressive attrition with each cell division. This forms the basis for the theory that the metered loss of telomeres can serve as a cellular mitotic clock that ultimately limits the number of cell divisions and cellular lifespan. In support of this view, shortening of telomere length with aging can be demonstrated in human peripheral blood cells,15,16,17 and the rate of shortening can be associated with conditions of increased hematopoietic stem cell turnover (e.g., in paroxysmal nocturnal hemoglobinuria).18
Many normal somatic human cells and differentiated tissues express readily detectable levels of the hTERC component. In contrast, hTERT expression and activity are more restricted because of stringent regulation on the levels of transcriptional initiation, alternative RNA processing, posttranslational modification, and subcellular localization. With the identification of an increasing number of TERT-associated proteins, it is likely that additional regulatory mechanisms will surface, such as those governing the accessibility of the telomerase holoenzyme onto the telomere end.19 Here again, a more complete elucidation of these regulatory mechanisms may provide additional therapeutic strategies that can preferentially target telomerase-mediated telomere maintenance in cancer cells. Indeed, the development of such selective strategies may become paramount and more challenging as recent studies have revealed low telomerase levels in cycling somatic human cells that were previously thought to have no telomerase activity.20 Eradication of residual telomerase function in these primary cells alters the maintenance of the 3′ single-strand telomeric overhang without changing the rate of overall telomere shortening, resulting in diminished proliferation rates and overall reduction in proliferative capacity. These studies support an additional protective function of telomerase at the telomeres21 and raise concerns that generalized antitelomerase therapy could lead to the immediate uncapping of telomeres in normal cells, thus limiting the use of antitelomerase therapy in cancer patients.
Lastly, in addition to forming the telomerase holoenzyme complex with TERC, TERT was recently shown to be able to interact with the RNA component of mitochondrial RNA processing endoribonuclease (RMRP). This distinct TERT/RMRP ribonucleoprotein complex has RNA-dependent RNA polymerase activity and produces double-stranded RNAs that can be processed into small interfering RNAs.22 Also, there is compelling experimental evidence that TERT can interact and engage the Wnt signaling pathway.23,24 These results suggest that TERT contributes to cell physiology independently of its ability to elongate telomeres, a fact that further complicates efforts to specifically target telomerase enzymatic activity as an anticancer therapy.
SENESCENCE
Primary human cells, even when cultured under optimal conditions, will eventually encounter a cell division barrier, termed cellular senescence, triggered by critically shortened telomeres. Senescence is a specific cell biologic phenotype composed of a permanent and durable growth
arrest, alterations in cellular morphology, expression of characteristic markers of senescence such as senescence-associated (SA) β-galactosidase activity, and alterations of chromatin structure to a growth-repressive state.25 Induction of senescence is intimately associated with p16INK4a and p53 activation, and when induced in vitro as a result of telomere dysfunction, this barrier is termed the Hayflick limit (M1) in honor of the discoverer of senescence.26 Because loss of p16INK4a-RB and/or p53 pathway function in primary human cells permits additional cell divisions beyond the Hayflick limit, these pathways appear to be involved in the activation of this senescence program brought about by the “shortened telomere” signal.
arrest, alterations in cellular morphology, expression of characteristic markers of senescence such as senescence-associated (SA) β-galactosidase activity, and alterations of chromatin structure to a growth-repressive state.25 Induction of senescence is intimately associated with p16INK4a and p53 activation, and when induced in vitro as a result of telomere dysfunction, this barrier is termed the Hayflick limit (M1) in honor of the discoverer of senescence.26 Because loss of p16INK4a-RB and/or p53 pathway function in primary human cells permits additional cell divisions beyond the Hayflick limit, these pathways appear to be involved in the activation of this senescence program brought about by the “shortened telomere” signal.
Beyond the connection with telomeres, cellular senescence appears to be a general anticancer mechanism, induced by a variety of oncogenic stresses. In addition to telomere erosion or structural uncapping (see later discussion), senescence is also induced by forms of DNA damage, oxidative stress, suboptimal growth conditions, and activation of certain oncogenes (reviewed in refs. 8 and 13). Senescence requires activation of the Rb and/or p53 protein; and expression of their regulators such as p16INK4a, p21CIP, and ARF (Fig. 4.2).27,28,29,30 An important form of senescence is induced by p53, which has several antiproliferative activities including stimulation of the expression of p21CIP, a cyclin-dependent kinase inhibitor. These inhibit progression through the cell cycle by inhibiting cyclin-dependent kinases that phosphorylate and thereby inactivate Rb and related proteins p107 and p130.31 The activation of p53 is predominantly effected by specific posttranslational modifications and its stabilization, which are prompted by the same stimuli that induce its expression, including telomere dysfunction, DNA damage, and oncogene activation (reviewed in refs. 18 through 20), as well as inappropriate cell cycle entry.32,33 A major sensor of oncogene activation and inappropriate cell cycle entry is ARF (also designated p14ARF in the human or p19ARF in the mouse), which binds to and blocks MDM2-mediated degradation of p53.33,34,35,36
Another prominent molecular correlate of senescence is up-regulation of the cyclin-dependent kinase inhibitor, p16INK4a, which increases markedly in senescent cells on passage in culture or advancing age in tissues.37 Correspondingly, ectopic expression of p16INK4a is sufficient to induce senescence in some cell types,38 and senescence can be delayed or prevented in some cell types by p16INK4a silencing or neutralization by antisense or siRNA.39,40,41,42,43 The regulation of p16INK4a is not as well understood as that of p53, although it appears to be induced by several stimuli, including oncogene activation and growth in culture.37 Activation of p53 (and hence p21CIP1) and/or accumulating levels of p16INK4a is able to produce Rb-family member protein hypophosphorylation and activation, which leads to repression of cellcycle progression,27,30 enabling initiation of the senescence process. Recent data have suggested that Rb may be of particular importance in the promotion of senescence compared with its related family members p107 and p130, likely explaining the frequent inactivation of Rb in human cancers relative to the other Rb-family members.44
Senescence as a Cancer Prevention Mechanism
Several lines of evidence have suggested an important role for senescence in the prevention of cancer in vivo. It is important to note that the field has been limited by the lack of robust in vivo biomarkers of
senescence. Although (SA)-β-galactosidase and p16INK4a expression have been used as markers of in vivo senescence, both have certain limitations and neither can be considered unequivocal proof of senescent state in vivo. These technical shortcomings notwithstanding, a growth arrest important for the prevention of tumorigenesis with characteristic features of senescence (p16INK4a expression and (SA)-β-galactosidase expression) has been noted in several murine and human in vivo tumor systems, and we believe the data suggest bona fide senescence occurs in the intact organism.
senescence. Although (SA)-β-galactosidase and p16INK4a expression have been used as markers of in vivo senescence, both have certain limitations and neither can be considered unequivocal proof of senescent state in vivo. These technical shortcomings notwithstanding, a growth arrest important for the prevention of tumorigenesis with characteristic features of senescence (p16INK4a expression and (SA)-β-galactosidase expression) has been noted in several murine and human in vivo tumor systems, and we believe the data suggest bona fide senescence occurs in the intact organism.
The lines of evidence for senescence as a tumor suppressor mechanism are quite strong. First, the aforementioned minimal residual disease data showing frequent oncogenic translocations and other mutagenic events demonstrate a constant need for tumor suppression, even in young animals. Additionally, several of the initially described “tumor suppressor” proteins that are mutated in familial cancer syndromes (e.g., p16INK4a, p53, Rb) are intimately involved in the induction of senescence. Mice lacking p16INK4a or p53 are prone to spontaneous cancers,45,46,47 and mice with severe compromise of the senescence pathway due to combined p16INK4a and p53 inactivation die of cancer, often harboring multiple synchronous primary tumors, with a median age of 8 weeks (compared with a normal murine lifespan of more than 100 weeks).19 Importantly, mice and humans with impaired p16INK4a and/or p53 function develop with only modest phenotypic alterations other than an age-dependent increase in cancer and an increased susceptibility to cancer following carcinogen exposure. Several groups have demonstrated a senescencelike growth arrest in murine and human tissues in association with somatic oncogenic events.48,49,50,51
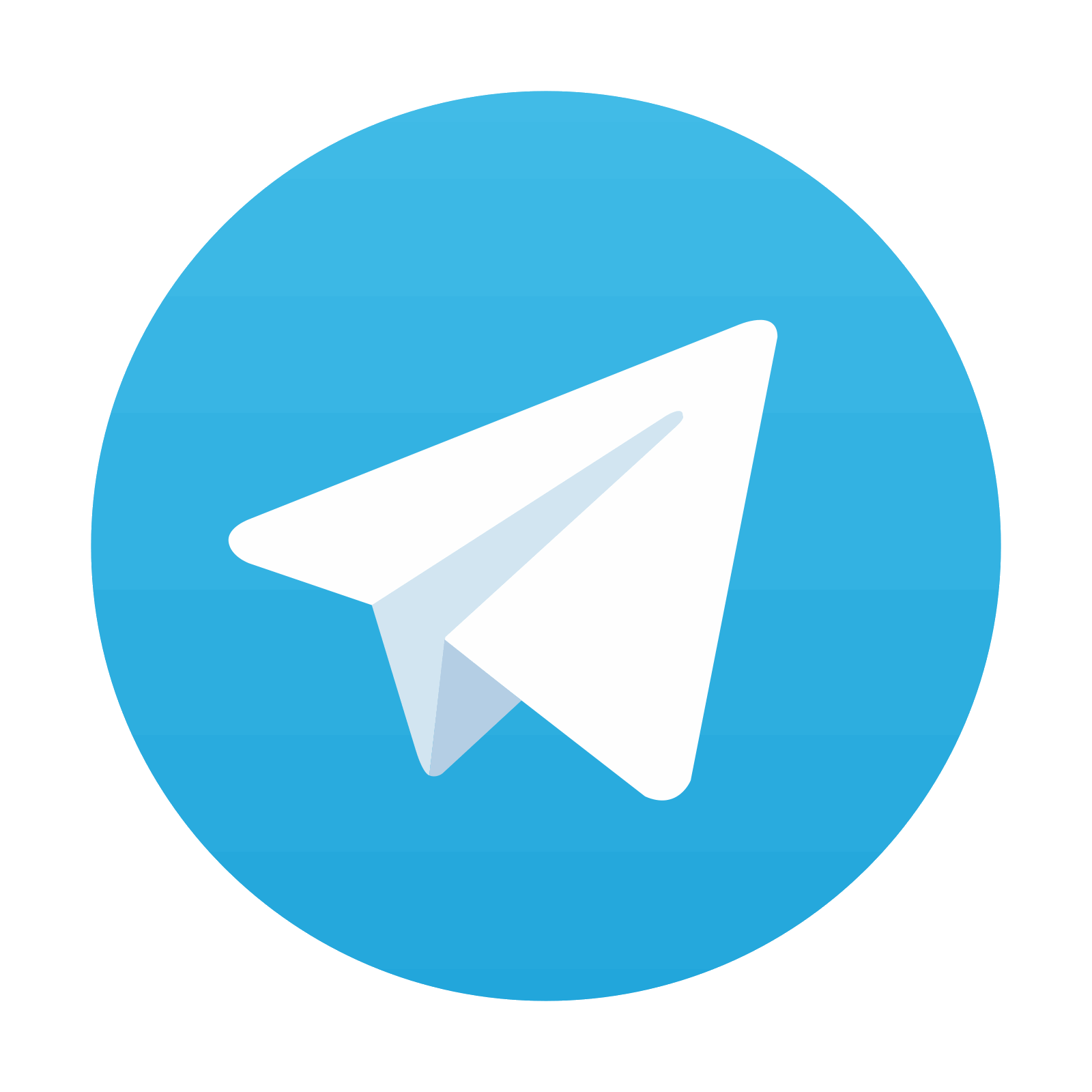
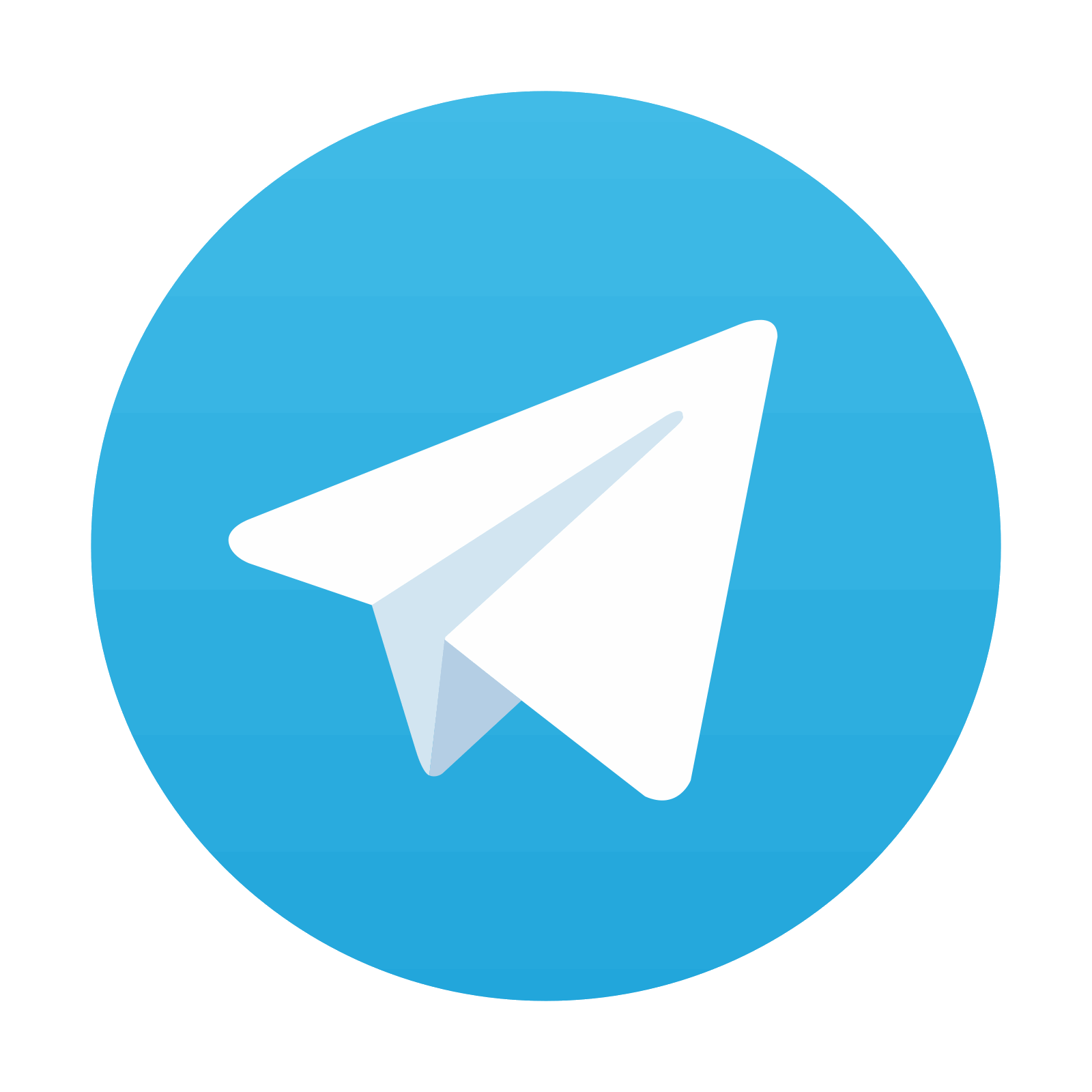
Stay updated, free articles. Join our Telegram channel
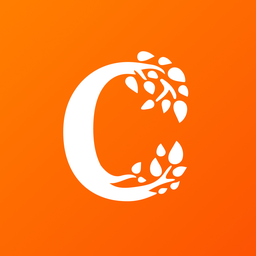
Full access? Get Clinical Tree
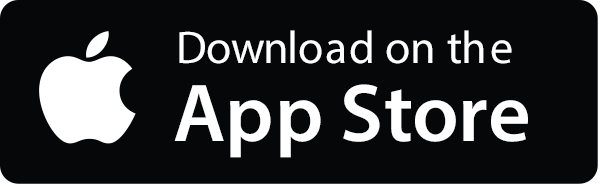
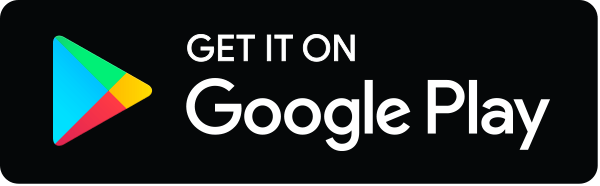
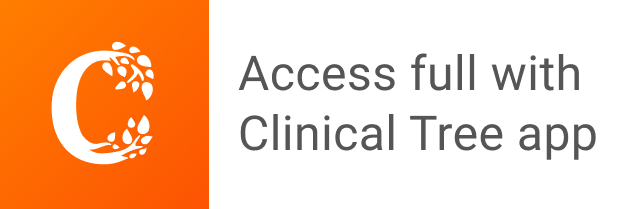