Smart Radiotherapy
When assigned this chapter title, “Smart Radiotherapy,” we were challenged as to the best approach. Looking through the previous edition, there was no precedent for a chapter with this name. Was there to be a companion chapter or chapters of “So-So Radiotherapy” or “Oy-Vay Radiotherapy”? Although one could perhaps all-too-readily envision examples, we decided that the most positive approach would be to enhance what will be an excellent and complete textbook by top quality experts by providing some of the new areas of research that might impact clinical radiation therapy over the next 5 years from our perspective in the National Cancer Institute (NCI), Division of Cancer Treatment and Diagnosis, Radiation Research Program. We anticipate that the radiation biology chapter will include molecular aspects of radiation therapy and the basic aspects of tumor microenvironment, the physics chapter will discuss particle therapy including protons and carbon ions, and the clinical chapters will include molecular characterization of tumors, the use of molecular imaging, and the current studies with molecular targeted agents.
So, taking a broad view, what is likely to impact radiation oncology practice and thinking over the next 5 or so years? The technology of radiation therapy is growing in complexity and cost so that clinical utilization and possibly reimbursement will require definite or at least reasonable proof of principle. Bringing the spectacular particle-therapy treatment plans seen on a computer screen to accurate and reproducible delivery to the patient requires understanding the pitfalls of dose distribution. Conceivably, new accelerator design may provide innovative and less-expensive technology and also a range of particles beyond protons. The rapid advance in cancer biology and the availability of many new molecular-targeted agents will require more rapid clinical trials, with the randomized phase II trial becoming a major tool, potentially using new clinical trial design techniques.1 The tumor microenvironment, long a province of radiation biology and radiation therapy, has enormous potential as a therapeutic target for cell killing and also for immune modulation. The threat of radiological terrorism has led to new investment in agents to mitigate radiation injury. This is research and development support above and beyond the NCI and National Institutes of Health funding allocation and may also have applications in cancer care. Nanotechnology and smaller biological delivery vehicles are bringing targeted systemic radiotherapy to a new level of possibility.
Cells know that they are irradiated, and as the “bystander effect” field has demonstrated, they not only respond but they tell their neighbors and progeny. Thus, the changing phenotype of the survivors might be utilized for treatment. Finally, on the macro level, the incidences of the noncommunicable diseases, cancer and heart disease, are already exceeding infectious diseases2,3 as a cause of morbidity, mortality, and economic disaster in developing countries. Smart and socially responsible radiotherapy needs to aim to reach the patients who can afford and access the very sophisticated expert centers and also those patients struggling to obtain care—albeit symptom relief or curative treatment—in an underserved community in the developing and developed world.
In that any textbook is somewhat out of date by the time it goes from writing to printing, rapidly changing research fields will be obsolescent almost from the time the chapter is written. Our aim in this chapter is to address the topics from a mostly conceptual viewpoint, including a few key references and examples. We are hopeful that these topics will provide the reader with a sense of optimism of the potential advances and contributions radiation oncology, biology, and physics and models of social outreach can make to innovative, effective, and far-reaching improvements in cancer care.
TUMOR MICROENVIRONMENT
The efficacy of radiotherapy is in part determined by tumor microenvironmental factors, including the extent of tumor oxygenation, the tumor pH, and the interaction of tumor cells with host stroma and with inflammatory cells. Tumors contain areas of poor vascular perfusion, low pH, and oxygen, which may provide regions in which the delivery and activity of cytotoxic therapies is attenuated.4 Resistance to therapy in these regions may be due to the effects of low oxygen, low pH, limited drug delivery presence of resistant cell niches,5 or a combination of all four. The tumor microenvironment influences, and is in turn influenced by, the interaction of tumor cells with host stroma and with inflammatory cells. In addition, certain tumor environments may select for more aggressive or resistant tumor clonogens.6
Changes in the tumor microenvironment occur during radiotherapy.7 Fractionated radiotherapy results in reoxygenation of the tumor due to elimination of well-oxygenated cells, thus increasing oxygen delivery to the previously hypoxic areas. Conversely, high-dose single fractions (over 13 Gy) have been shown to result in destruction of both tumor cells and vascular endothelium.8 How and under what circumstances radiation-induced vascular injury impacts tumor responses continues to be a topic of investigation and is relevant to the current use of large fraction stereotactic radiosurgery or therapy.9,10,11,12
Tumor Hypoxia
Perhaps the best-studied example of tumor microenvironment effects on cancer cell survival is tumor oxygenation. It is known that radiation under atmospheric oxygen conditions enhances killing by a factor of 2 to 3 compared to irradiation under anoxic conditions, which has been termed the oxygen enhancement ratio13 (as reviewed by Horsman et al.7). In the clinical setting, hypoxia affects outcome after radiotherapy.14–15,16 But hypoxia has also been shown to be a poor prognostic factor after chemotherapy17 and surgery.18,19 These findings were attributed to chronic hypoxia induced under controlled culture conditions or oxygen levels measured with electrodes or immunohistochemical staining. Overcoming hypoxia by targeting hypoxic regions in tumors has proven difficult. Recent advances in noninvasive tumor hypoxia imaging may come into play in the future, allowing precise definition of hypoxic tumors and tumor subregions through imaging with positron emission tomography (PET), magnetic resonance imaging (MRI), or electron paramagnetic resonance oxygen imaging.20–23
More recent studies have shown that acute fluctuations in tumor oxygenation (“transient” or “cycling” hypoxia) interspersed with periods of reoxygenation may be even more effective in promoting tumor survival and progression than chronic hypoxia.7 Defining whether intermittent or chronic hypoxia is more radiobiologically important is at present an open question; however, it is likely that both contribute to resistance. It is clear that cycling hypoxia may complicate efforts to identify and target radiobiologically important hypoxic regions within tumors using approaches such as radiation dose painting to hypoxic tumor subregions.24 Regions of cycling hypoxia may be more difficult to image using current methods, such as nitroimidazole markers, which are better suited to detecting chronic hypoxia. Developments in imaging with MRI and electron paramagnetic resonance may facilitate real-time detection of hypoxic transients in the future.
Hypoxic Cell Sensitizers and Cytotoxins
There are two chemical approaches used to reduce the impact of hypoxic cells on radiotherapy response: radiosensitization and hypoxic cell cytotoxins. The nitroimidazole hypoxic cell sensitizer, nimorazole, used with radiotherapy has shown promising results in patients with hypoxic tumors.25,26 However, testing and adoption of this drug in countries other than Denmark have been slow at least in part due to a lack of patent protection for the drug (an issue that may be relevant to the use of chemotherapeutic agents as radiation sensitizers). General adoption of this treatment could, in theory, improve outcome in certain tumors such as head and neck squamous cell carcinomas. An alternative approach of using hypoxic cell cytotoxins has also been tested. Tirapazamine (TPZ, 3-amino-1,2,4-benzotriazine-1, 4-dioxide) undergoes reduction under hypoxia to form a highly reactive radical that causes DNA damage leading to cell killing.27 Under normoxic conditions the reduction of TPZ is reversed, thus providing the specificity of cytotoxicity for hypoxic tissues.28,29 Despite promising early results, a recent phase III trial of TPZ in combination with chemoradiotherapy failed to demonstrate an improvement in failure-free survival, time to locoregional failure, or quality of life among patients with head and neck cancers.30 The reasons for this failure are the subject of debate,31,32 and the future of this compound is at present unclear. Development of new generation hypoxic cell cytotoxins with greater preclinical efficacy than TPZ is under way.32
The identification and selective treatment of hypoxic tumors is likely, in the future, to enhance the results of radiotherapy. The failure to select patients with hypoxic tumors in past studies may have led to the negative results with many of the approaches tested to date, but novel imaging methods to identify hypoxia and new generation hypoxic cytotoxins and sensitizers will benefit this approach.
Tumor Angiogenesis
Normal angiogenesis is a highly regulated process involving endothelial cells with differing functions (tip, stalk, and phalanx cells) under tight regulation of oxygen sensors (prolyl-hydroxylase-2 [PHD-2]), growth and maturation factors (vascular endothelial growth factor [VEGF], angiopoetins), and transmembrane receptors (Notch family) (reviewed in Carmeliet et al.33). Small changes or imbalance in the expression of these regulators can have a significant impact on vascular development and lead to the formation of abnormal tumor vessels that are inefficient in the transport of nutrients, oxygen, and metabolic wastes. Limited perfusion and convection also reduce the efficacy of chemotherapeutic delivery. Vascular endothelium largely comprises host cells, and these cells, unlike tumor cells, are genetically stable and thus an attractive target. Antiangiogenic agents reduce or cut off tumor vascular flow by selective targeting of tumor vessel growth by blocking VEGF signaling. Targeting the tumor vasculature was originally proposed by Judah Folkman.34 It is now clear, however, that this approach is complicated by the fact that development, structure, and the potential for recovery of tumor vasculature after treatment are influenced by bone marrow–derived progenitor cells in addition to the tumor and other stromal elements.35 In addition, where tumor cell–derived vascular channeling (vasculogenic mimicry) plays a role in tumor circulation,36–38 the effects of antiangiogenic strategies that target host vascular endothelial cells may not be as effective. The activity of cilengitide, an inhibitor of integrin binding to extracellular matrix, as an antiangiogenic agent39 is of particular interest in this regard. The resistance of glioblastomas to other antiangiogenic therapies may be related to their potential for vasculogenic mimicry.38,40 As cilengitide targets integrins rather than endothelial cells per se, it might be active against vessel structures derived from tumor rather than host endothelial cells.
VEGF can be induced in response to radiation, and inhibition of VEGF can increase tumor control after radiation in preclinical models.41 VEGF receptor inhibition reduces endothelial cell proliferation in vitro after irradiation and also reduces microvessel density in irradiated tumors.42 Clinical trials have suggested a potential benefit in combining radiation and antiangiogenic therapy in rectal cancer and sarcoma.43,44 However, the toxicity of bevacizumab in combination with radiotherapy has been of concern in some tumors.45
Tumor Vessel Normalization
Vascular normalization is a term coined to denote transient changes observed in tumor vessels after VEGF inhibition with DC101, a VEGF receptor-2 antibody.46 Other antiangiogenic approaches have shown similar effects on tumor vasculature. Methylselenocysteine, which has antiangiogenic activity, has been shown to induce a more mature morphology in FaDu xenograft tumor vessels, accompanied by enhanced perfusion and a fourfold increase in doxorubicin delivery to treated tumors.47 Normalization appears morphologically as a reduction in the chaotic branching and tortuosity of tumor vessels and is accompanied by a reduction in tumor hypoxia and enhanced radiosensitivity. The effects of vascular normalization may also involve reduction of tumor interstitial fluid pressure. High tumor interstitial fluid pressure has been shown to impact survival after irradiation and may also be influenced by the tumor stroma.48 Tong et al.49 have shown that blocking VEGF signaling by DC101 results in decreased interstitial fluid pressure in tumor xenografts in mice. Although the normalization period offers an opportunity for improved response to radiation and chemotherapy, the period of normalization obtained with direct targeting of VEGF signaling in preclinical studies lasts less than a week, followed by vascular insufficiency due to strong antiangiogenic activity of these inhibitors. In addition, vascular normalization may not occur in all tumors and has not been observed in all studies (reviewed in Horsman and Siemann50).
In the clinical setting, vascular normalization has been proposed as a predictive marker in patients undergoing cediranib treatment for recurrent glioblastoma.51 Elevated blood perfusion after cediranib treatment has recently been associated with increased survival in these patients.52
More durable normalization of tumor vasculature might be clinically achievable through growth factor or oncogenic signaling inhibition. Studies have shown changes in vascular morphology, function, and maturation accompanied by decreased tumor hypoxia after inhibition targeting epidermal growth factor (EGF) receptor, RAS, phosphoinositide (PI) 3-kinase, or AKT in human tumor xenografts and spontaneous mouse tumors that last up to two weeks.53 Others have shown showed that blocking EGF signaling increased tumor uptake of cisplatin at the same time that enhanced vascular function and oxygenation were seen in tumor xenografts.54 Signaling inhibition in these studies decreased, but did not eliminate, VEGF production by tumors, which might account for the effects seen and their duration.
Other approaches to altering tumor vasculature are being investigated. Inhibition of α(v)β(3)/α(v)β(5) integrin has been reported to cause effects similar to signaling inhibition on tumor vascular morphology.55 Using another approach, investigators showed that shutting off NOS1 production by U87 glioblastoma tumors restored a nitric oxide gradient around the tumor vessels. This was accompanied by a normalized phenotype with less tortuous and more abundant vessels that had increased perivascular cell coverage.56 Hypoxia in these tumors was reduced and their response to radiation increased as measured both by relative tumor volume and survival endpoints. Modulation of host PHD-2 can affect tumor vascular structure and maturity.57 Inhibitors of PHD-2, such as DMOG, might be developed for clinical application.
Inhibition of Vasculogenesis
Vasculogenesis, unlike angiogenesis, recruits cells from distant sites, including the bone marrow, to participate in the formation of new blood vessels (reviewed in Patenaude et al.58). The process is thought to be an important contributor to the tumor vasculature, although the precise contribution of the different recruited cell types is still a matter of debate.35 The CD11b positive, matrix metalloproteinase-9 (MMP-9) expressing myeloid cells appear to be central to this process.59 A neutralizing antibody to CD11b inhibited the recruitment of myeloid cells to tumors and prolonged radiation-induced regrowth delay. Additionally, mice with reduced CD11b expression showed enhanced xenograft radiosensitivity.60 Stromal derived factor-1 (SDF-1) and the chemokine receptor CXCR4 also contribute to recruitment of endothelial cell precursors and could be targeted. Inhibition of the CXCR4/SDF-1 interaction reduced bone marrow–derived progenitor cell recruitment into irradiated glioblastoma orthotopic xenografts and slowed tumor regrowth.61 An inhibitor of SDF-1, AMD3100, is in clinical use for stem cell mobilization and could be quickly adopted for this purpose. Inhibition of tumor vasculogenesis may be an important future approach to block tumor vascular recovery after treatment.
Antivascular Therapy
Selectively destroying tumor vasculature with vascular disrupting agents (VDA) is an approach that seeks to kill tumors by shutting off their blood supplies (reviewed in McKeage and Baguley62). This approach, however, acts primarily on the tumor core and does not eradicate host vessels that border the tumor and are in proximity of normal host vasculature. Tumor cells at the host–tumor interface can remain viable after this treatment to repopulate the tumor. Furthermore, vascular disruption could generate areas of hypoxia that still contain viable tumor cells, thereby selecting for more resistant clones.6 Combining VDA treatment with a cytotoxic modality could overcome this problem. The effects of VDAs both alone and in combination with antiangiogenics, chemotherapy, radiation, or other modalities, have been examined in a number of preclinical studies (reviewed by Horsman and Siemann50). VDAs are currently in phase II to III clinical trials alone or in combination with cytotoxic chemotherapies.62–64
Tumor Stroma and Extracellular Matrix
The tumor stromal compartment, including the extracellular matrix, host fibroblasts, and immune cells, is known to contribute to tumor progression and survival.65,66 In certain tumors, like pancreatic cancers, activation of quiescent stromal cells, known as stellate cells, to myofibroblast-like cells involved in extracellular matrix production contributes significantly to tumor progression.67 Activated fibroblasts deposit collagens, leading to changes in stromal rigidity. This mechanism for stromal-induced tumor promotion includes intercellular signaling initiation via integrins to prosurvival pathways, including PI3-kinase.68 Stroma-mediated change in tumor tensile properties is thought to contribute to tumor progression.69
There are multiple points at which stromal changes can be inhibited, including inhibition of fibroblast or stellate cell activation by angiotensin-converting enzyme (ACE) inhibitors or other antifibrogenic compounds such as halofuginone.70 Signaling by the cytokine tumor growth factor-β (TGF-β) strongly promotes fibrosis, both in response to tumor growth and in normal tissues in response to treatment with radiation. Direct inhibition of TGF-β in tumors can interrupt or mitigate desmoplasia and reduce tumor growth.71 Angiotensin-II (Ang II) can promote stellate cell growth under pathologic conditions, and ACE inhibitors act in part through blocking Ang II.72 Angiotensin-(1-7), an endogenous 7 amino-acid peptide antagonist of Ang II, has been shown to reduce TGF-β expression, block proliferation of cancer-associated fibroblasts, and inhibit tumor growth.73 Inhibition of Hedgehog pathway signaling results in transient enhancement of vascular perfusion and gemcitabine delivery to spontaneous pancreatic cancer in a mouse model.74 This model shares a high degree of stromal cell activation and poor vascular perfusion with human pancreatic ductal adenocarcinomas. In these tumors, the downstream Gli transcription factor is activated in the tumor stromal cells, resulting in a desmoplastic reaction. Inhibition of Hedgehog signaling to the Smoothened receptor blocks activation of Gli and thereby reduces the activation and proliferation of the stromal compartment.
Integrin binding and signaling have been identified as a potential target for cancer treatment, as these receptors are involved in both tumor growth and angiogenesis (reviewed in Desgrosellier and Cheresh39). Cilengitide is a pentapeptide mimic of the RGD binding site that blocks α(v)β3 and α(v)β(5), binding to extracellular matrix components.75 In addition to its antiangiogenic activity, it inhibits focal adhesion kinase signaling.76 Cilengitide is well tolerated and has some antitumor activity as a single agent.77 It has been shown to be active in combination with radiation in several, but not in all reports.78–80 However, questions remain about potential normal tissue toxicity in combined modality treatment because integrin signaling is required for normal cell function and survival.79 In this regard, cilengitide was shown to aggravate experimental liver fibrosis in an animal model.81 Despite this, clinical trial results combining cilengitide with temozolomide and radiation have been encouraging82 when compared to historical controls. The primary focus of cilengitide studies has been on glioblastoma, but other tumor targets including melanoma and breast and pancreatic cancers are being tested for sensitivity to this agent.78,83,84
Targeting Stem Cells
The cancer stem cells (CSCs) model is currently used to as a framework for understanding cancer proliferation, growth and treatment resistance (reviewed in Bednar and Simeone85). CSCs are thought to be a subset of cells at the peak of a developmental chain within a tumor with the capacity for unlimited self-renewal and were first identified in breast cancer as cells that were surface marker CD44+/CD24– and capable of tumor initiation.86 These cells give rise to more differentiated daughter cells with less replicative and tumorigenic potential that make up the bulk of the tumor cell population. The CSC may be more quiescent than the general population of cells in a tumor and relatively resistant to cytotoxic therapy.87 Some studies have identified perivascular niches for these cells (reviewed in Borovski et al.88) and the conditions that promote their colonization and survival,5 but the identification of both the stem cells and their niches in a disorganized and dynamic tumor environment remains challenging.89 The perivascular niche and, in particular, the endothelial cells may both aid in tissue repair and promote tumor growth by secreting “angiocrine factors.”90 Targeting these factors could be an approach to cancer treatment. Disruption of the perivascular niche with vascular disrupting agents or antiangiogenics might also reduce CSC numbers.
The pericyte component of the tumor vasculature is another potential therapeutic target. Interferon-b treatment has been shown to decrease CD34+ cancer stem cells in a U87 orthotopic glioblastoma tumor model. Examination of the perivascular niches in which these stem cells are found showed increased numbers of pericytes. The increase in pericytes is hypothesized to block interactions of glioma stem cells with the vascular endothelium and also, perhaps, reduce their exposure to growth factors,90 thus reducing stem cell frequency in these tumors.91 The potential for CSCs as targets and potential biomarkers for radiotherapy has recently been reviewed by Krause et al.92
Immunological and Inflammatory Responses
Immune responses can both be modulated by and contribute to radiotherapy treatment responses (reviewed in Shiao and Coussens93). Although whole-body radiotherapy is immunosuppressive, localized treatment may boost immune reactivity toward a tumor by enhancing antigen presentation. This may be particularly true in the case of high-dose hypofractionated, but not single dose, delivery, as discussed below.94 Irradiation can promote tumor cytokine release, as well as increasing class I antigen expression and intercellular and matrix cell surface adhesion molecules including ICAM-1 E-selectin and VCAM-1.93 Manipulations of the immune system and tumor infiltrating cells are also being tested as therapeutic approaches. In the future, radiotherapy may be combined as an adjuvant to vaccination to promote antitumor immune responses.95 As mentioned above, inhibition of CD11b monocytes can inhibit tumor revascularization.60 Conversely, tumor hypoxia itself may have an immunosuppressive effect.96 Tumor-associated macrophages (type M2) are known to influence both progression and metastasis. Their presence is associated with poor prognosis.97,98 Modulating the differentiation of macrophage precursors to shift the balance toward effector (type I) macrophages may prove to be additional strategy for intervention.
Recent work indicates a novel potential target within the tumor stroma, fibroblast activation protein, which has the potential to enhance innate antitumor immune responses. In a transgenic mouse model the elimination (through genetic manipulation) of fibroblasts and pericytes expressing fibroblast activation protein enhanced antitumor immune responses. Activation of tumor-infiltrating T cells and the resulting increase in interferon-g and tumor necrosis factor-α (TNF-α) promoted the antitumor immune response in a transgenic mouse model.99 A possible pharmacologic approach to elimination of these activated fibroblasts is by inhibition of the matrix enzyme lysyl oxidase-like-2 (LOXL-2). Inhibition of this enzyme using a monoclonal antibody decreased desmoplasia and both primary and metastatic tumor growth100; small molecule inhibitors of this enzyme also exist. Targeting lysyl oxidase (LOX), which has been shown to promote tumor growth in orthotopic and spontaneous mouse tumor models, might also be a means of inhibiting tumor growth (Fig. 5.1).68
Summary of Microenvironment
It is not possible to predict exactly how radiotherapy will evolve in its utilization of knowledge regarding the tumor microenvironment and host–tumor interactions. What appears clear is that increasing emphasis will be placed on these factors. Targeting changes specific to the microenvironment has the advantage of being largely tumor specific and potentially less toxic than cytocidal therapies. Future developments may allow for hypoxic tumor region dose painting. Alternatively, hypoxia targeting drugs may finally reach clinical maturity. Inhibition of hypoxia in tumors may also arise from strategies that revert tumor vasculature to a more normal morphology and function. This strategy has the added advantage of reducing hypoxia selection for aggressive tumor clones and enhancing chemotherapy delivery. The impact of the immune response on tumors, and in turn the effects of different radiotherapeutic interventions on immunity, may soon be exploited. Findings relating to the impact of host stromal elements on tumor cell radiation survival through direct intercellular signaling, autocrine stimulation, or mechanical force may also soon reveal new targets and interventions applicable to radiotherapy.
Combining agents that alter the tumor environment with radiotherapy requires strong preclinical data demonstrating at least additive effect and acceptable toxicities. Careful preclinical testing, including normal tissue response evaluation, may avoid subsequent problems in many instances. Having markers, and imaging, that detect the targets of interventions, such as hypoxia, cancer-associated fibroblasts, or immune infiltrating cells, and that can be used to assess intervention-mediated changes in the environment will be critical to fully exploiting this approach.
FIGURE 5.1. The tumor response to radiation is influenced by a number of interacting microenvironmental factors, which may in the future serve as targets for clinical intervention. Micrograph of human a tumor xenograft stained for nuclei (blue), vasculature (green), and hypoxia (red). (Courtesy of Professor A. van der Kogel.)
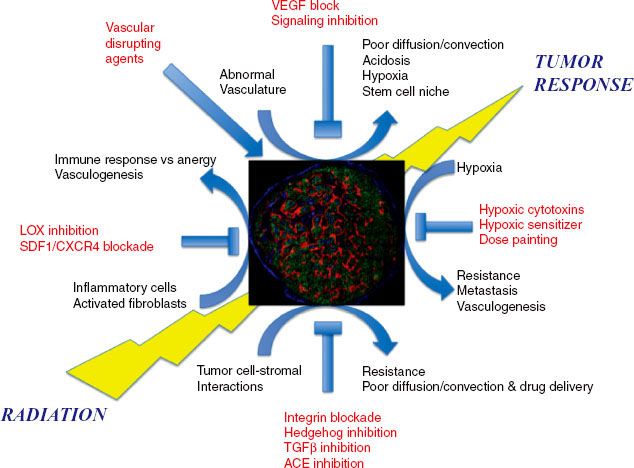
INNOVATIVE TARGETING
Molecular Imaging
To date, advances in radiotherapy (RT) have followed progress in nuclear and particle physics, computer sciences, and imaging technology combined with clinically relevant modifications of fractionation schemes resulting from radiobiological research at the laboratory and clinical levels. Because of the physical limitations of radiation dose delivery, further technical improvements are likely to provide only incremental benefits. Moreover, even the most advanced approaches to treatment planning, including sophisticated modeling response of normal and neoplastic tissues to radiation, in general, do not consider the specific characteristics of individual tumors, let alone nonoperable metastatic lesions. For these reasons, further increases in tumor control will require a better understanding of the mechanisms involved in cellular responses to radiation and their possible activation in individual tumors.
One cannot overemphasize the role of molecular imaging in the future of RT striving to individualize treatments, optimize responses, avoid toxicity and minimize the costs. Imaging of the molecular and biochemical features of cancer and their changes in response to therapeutic intervention can be sequentially repeated, allowing implementation of adoptive radiation therapy. Thereby, radiotherapy may extend the notion of personalized medicine from the current treatment planning stage to adoptive RT with molecular imaging, providing tools to monitor the effect of the therapy on the main tumor characteristics. For example, new more general markers of sustained proliferative signaling will be utilized including 18F-FDHT,101 18F-FLT,102 and growth factor receptor–specific tracers such as 18F-Affibody.103 Replicative potential will be visualized by direct telomerase imaging.104 Tumor-promoting, chronic inflammation can be observed by 18-fluorodeoxyglucose (18F-FDG)105 or NK1106 imaging. FDG-, FLT-, RDG-, and MMP-targeted radiotracers107 could be potentially used to observe changes of invasiveness and metastatic potential. RGD imaging of α(v)β(3)-intergin expression108 will provide data on changes in neoangiogenesis. Induction of apoptosis will be monitored by 18F-ML10 imaging.109 FDG will provide data on the deregulated cellular energetics. As discussed in the preceding section on tumor microenvironment, hypoxia creates resistance to radiation and induces an aggressive cancer phenotype.110 Therefore, the hypoxic parts of the tumor need to be exposed to a higher radiation dose or hypoxia-target agents. In the future, the presence of tumor hypoxia may be an indicator for the concomitant use of agents that target hypoxic pathways, a number of which are in development. Multiparametric analysis of tumor response and online adjustment of the RT based on the response of individual tumor will allow optimizing the treatment with carefully done clinical trials to assess the efficacy of the technological and biological intervention.111
Nuclear Medicine
Systemic targeted radiotherapy is an evolving and promising modality of cancer treatment. It provides a unique means to efficiently eradicate disseminated tumors cells and small metastases before they are detectable by currently available methods. The key characteristic of systemic targeted radiotherapy is its specificity. Using tumor-homing characteristics of a radioactive compound or conjugating therapeutic radionuclei with a tumor-targeting molecule (antibody, antibody fragment, or peptide), it can deliver higher amounts of a radionuclide to cancer cells than to normal tissue. A number of antigens and receptors present on the tumor cell surface, including CD20, CD45, PSMA, mucin-1 (MUC1), HER2, EGFR, TNF as well as VEGF, and α(v)β(3) abundant on the vascular endothelial cells within newly developed blood vessels have been advocated as potential targets for radioimmunotherapy (RIT) in patients.112
There are two different approaches that have been introduced into clinical practice, including the use of direct conjugation of radioisotope tagged to mAb or pretargeting of the tumor. In the first case, the patient receives a diagnostic dose of an antibody labeled with radionuclide compatible with appropriate imaging modality (single-photon emission computed tomography [SPECT] or PET). If the conjugate is stable and there is sufficient localization of an antibody at the site of disease, the patient can be injected with a therapeutic dose capable of inducing cytoreductive and potentially curative effects. This approach, however, has some limitations. First, radiation dose delivered to solid tumors might be insufficient due to poor penetration of the large size radioimmunoconjugate. Moreover, rather long serum half-life of mAbs together with long decay time of the radioisotope increase the radiation exposure to normal organs and can contribute to bone marrow toxicity.
In a pretargeting approach, the radionuclide is administrated separately from the antibody vehicle. There are two strategies: one involves the administration of radioactive biotin for selective localization on antibody streptavidin conjugates. This approach takes advantage of the rapid pharmacokinetics of the small biotin molecule and the high affinity of avidin-biotin binding.113 Alternatively, chelators of radioactive metals and multispecific antibodies that are capable of simultaneously binding to a tumor-associated antigen and a metal chelator could be used.114
Advances in imaging and radiation transport will allow new approaches to radionuclide therapy treatment planning. By administration of a dosimetric (trace-labeled) dose and determination of the patient’s residence time (a measure of how long the radionuclide is retained in the body), the therapeutic dose can be precisely adjusted to maximize the therapeutic effect and minimize toxicity. The paradigm of a targeted drug with a patient-specific dose may become more routine as targeted therapies are further developed along with better assays to directly measure drug levels. For the present, whole-body dosimetry is routinely applied for RIT, but in the near future the patient-specific maximally tolerated therapeutic radiation dose will be used to maximize efficacy while minimizing organ and bone marrow toxicity.111
Nanotechnology
In the future, the clinical application of nanotechnology might revolutionize cancer treatment. Multifunctional nanoparticles allow simultaneous targeted delivery of diagnostic and therapeutic agents to the tumor tissue, giving rise to a new, fast growing field of theragnostics that combines the modalities of diagnosis and therapy.115
Gold Nanoparticles
Over the past two decades, radiation dose enhancement by high atomic number elements such as iodine has been explored for cancer radiotherapy.116 As a small-molecule radiation dose enhancer (smRDE), iododeoxyuridine (IUdR) has been used due to its facile incorporation into cellular DNA.116,117 The subsequent external irradiation of high-energy photons on the IUdR-containing target cells can trigger the secondary emission of photoelectric radiation (i.e., Auger/secondary electron emission or x-ray fluorescence).118 The resulting triggered emission from smRDE can cleave the nuclear DNA double strands that can induce the radio-sensitized cell death.119 In this smRDE-mediated radiotherapy, several advantages have been demonstrated. The triggered emission from smRDEs generally decays in several millimeters, which is a typical length scale of a cell so that the resulting cytotoxicity is highly dependent on the cellular location of smRDEs. Therefore, only the smRDEs located inside the target cells can deliver high toxicity upon radiation while their off-target toxicity is reduced by virtue of their being outside the cells.120
Although such smRDEs can improve the radiotherapeutic efficacy with reduced side effects, safe and effective delivery of smRDE to target tissue remains one of the major drawbacks to clinical applications.120 As iodine-attached tumor-targeting antibodies were used to improve their biodistribution, their targeting and therapeutic efficacies were not satisfied due to the rapid dehalogenation mechanism in DNA as well as the heterogeneity and limited expression of target receptors on cancer cells.121 Furthermore, the prolonged treatments with high doses of iodine compounds should be avoided due to their toxic side effects to the host organs.
These limitations might be circumvented by development of a nano-encased RDE (nanoRDE) platform, based on functionalizable polymer-modified nanoparticles. NanoRDE platforms can demonstrate several advantages. First, the biodistribution of nanoRDE can be highly improved by the “enhanced permeation and retention” effect in solid tumor tissue, which allows for the selective accumulation of nanoRDE at diseased sites (passive targeting).122,123 Second, high amounts of nanoRDE can be readily internalized in target cells by endocytic pathways, which are known as a completely different cellular internalization mechanism from that of small molecules.124 In addition, the subsequent acidic endosomal environments can be used as a trigger for the pH-sensitive release of additional chemotherapeutic agents.125
In conventional chemotherapy, the rapid developments of multidrug-resistant characteristics in cancer cells cause a critical problem in clinical cancer treatments.95 As such, it is obvious that a combinational therapy is highly favorable for the complete remission of cancers rather than a single-type treatment. To this end, anticancer drug-conjugated nanoRDE would be “smart combined modality therapy” as a multimodal delivery platform for both radio- and chemotherapy.
Gold nanoparticles have been tested for improvement of both chemotherapy126 and radiotherapy.127 The TNF-α PEG-colloidal gold nanoparticle, CYT-6091 (Citimmune, Gaithersburg, MD), has been shown to selectively traffic to tumor tissue. This agent has been evaluated for its ability to selectively deliver TNF-α to tumors in clinical trials. Gold nanoparticles are available in a broad range of sizes.
In addition to its properties as a nano carrier, colloidal gold, being a high-Z element, may also increase the radiation dose delivered specifically to the target cells. In this system, gold nanoparticles (AuNPs) are used as inorganic nanoRDEs for radiotherapy as well as a delivery platform for chemotherapeutic agents. Due to the K-edge of gold at 80.7 keV, x-ray radiation with the energy level of Au K-edge can trigger the secondary emission of photoelectric radiation from AuNPs.128,129 The resulting radiation can induce the degradation of target molecules by ionization and can interact with surrounding water molecules to produce reactive oxygen species that can damage the target molecules.130 As such, AuNP-sensitized degradations of plasmid DNA131 and human proteins132 upon x-ray radiation have been demonstrated in in vitro model systems. Additionally, when AuNP-containing tumor cells were irradiated, increased apoptotic cell death was detected due to the continuous stress on cytosolic organelles.133 Furthermore, enhanced in vivo efficacy of AuNPs upon radiotherapy was also observed in human cancer-bearing mouse models.134 However, these AuNPs have shown very poor pharmacokinetic results due in part to their limited surface functionality as a bare colloidal particle.
Recently, AuNPs have been used in a wide range of biological applications due to their biocompatibility and well-known surface chemistry. Using the known reactions, AuNPs can be readily modified with thiol-end-capped polymers135 that can significantly alter the pharmacokinetics.136,137 This functional polymer can be prepared by highly controllable reversible addition-fragmentation chain-transfer radical polymerization, which allows for copolymerization of a wide range of monomers.138
As the gold K-edge is at 80.7 keV and the enhancement is optimal in the photoelectric-dominated x-ray spectrum, irradiation conditions could be optimized by using monoenergetic x-rays. Finally, gold can be easily activated with thermal neutrons to emit 411 keV γ-rays, which could provide a means for monitoring their biodistribution by SPECT.
Liposomes
Another alternative approach would be using radiation as a trigger releasing or activating drugs delivered to the tumor prior to irradiation. One option could provide radiosensitive liposomes. Liposomes have been explored as viable carriers for targeting, imaging, and delivery of payload of drugs for decades.139 However, practical applications of liposomes are limited due to poor understanding of in vivo interactions, factors affecting biodistribution, and lack of modalities for controlled disruption of liposomes at optimal time within a limited volume.140,141 To date, various triggering modalities such as local hyperthermia, pH-triggered, tissue-associated enzyme-triggered, and radiation-triggered drug release have been developed.139 Among these, electromagnetic radiation-triggered release of liposomal drugs appears a promising approach and includes strategically designed phospholipid molecules to initiate light-induced trigger. These liposomes are based on the principle of photopolymerization of lipids,142 photosensitization by membrane anchored hydrophobic probes,143 or photoisomerization144 of photo-reactive lipids. However, none of the formulations developed so far have been successful for in vivo applications, presumably due to the lack of adequate photon energy produced by the radiation source(s) or inability of radiation to penetrate into biological tissues (Fig. 5.2).
FIGURE 5.2. Three examples of combining nanotechnology with radiation therapy. A: Radiation triggers drug release from liposomes. B: Radiation prompts nanoscintilators to emit light that interacts with photosensitizes to produce toxic reactive oxygen species (photodynamic therapy). C: Nanoparticles are used to deliver chemical elements with a high atomic number that, after being internalized by tumor cells, enhance the effectiveness of x-rays.
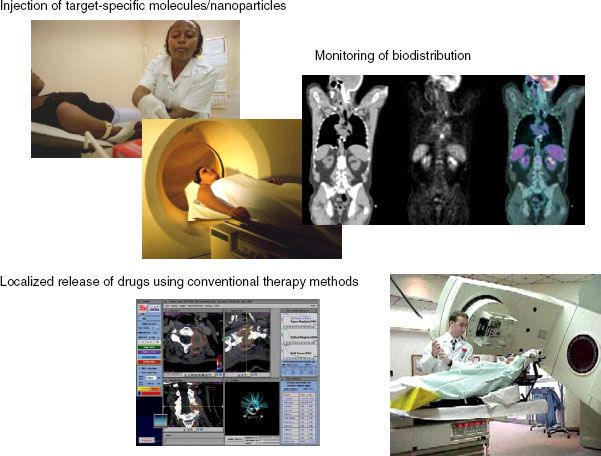
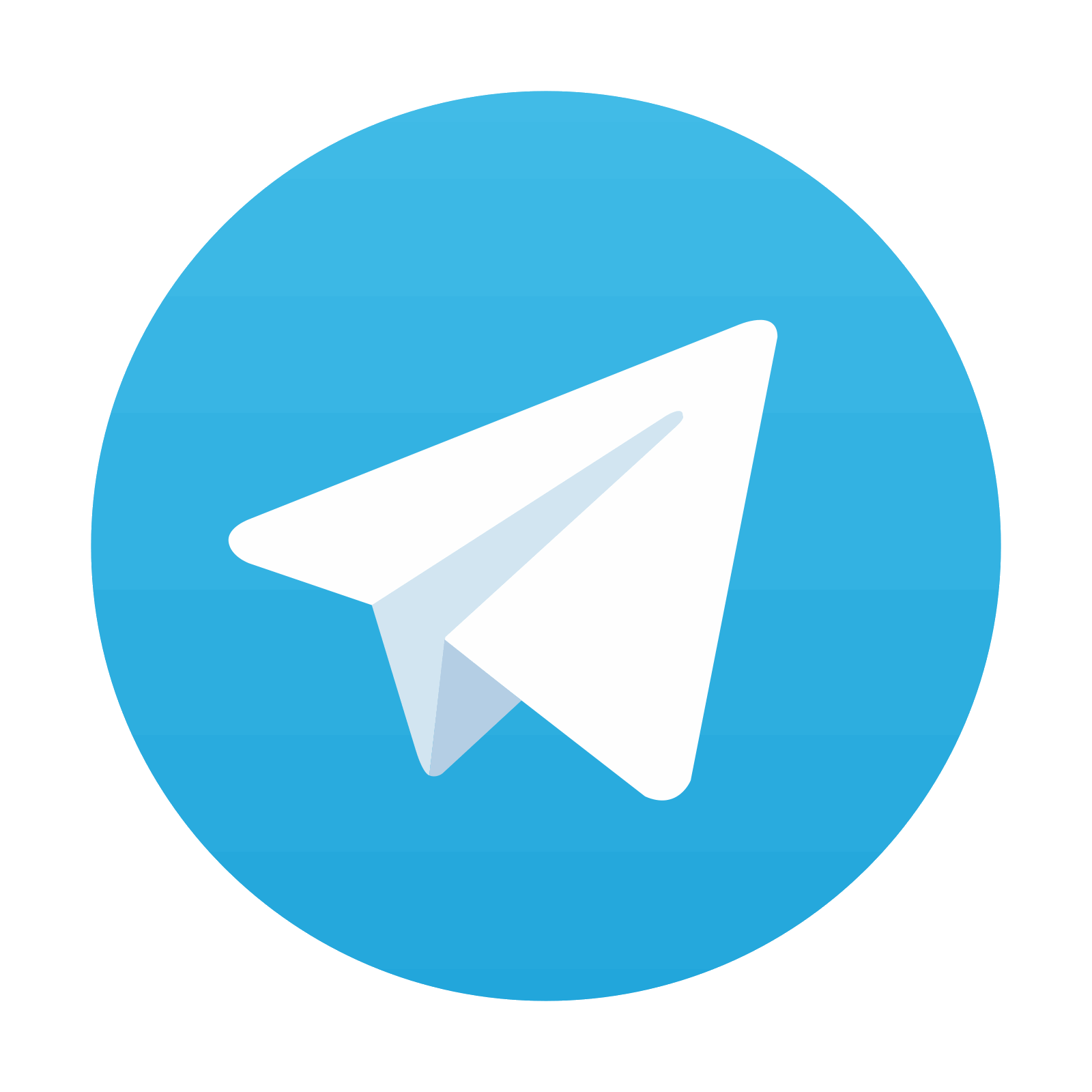