Shear Effects on Platelets and the Vessel Wall in the Pathogenesis of Atherothrombosis
David L. Bark Jr.
Warwick S. Nesbitt
Angus K.T. Wong
Shaun P. Jackson
The hemodynamic effects of blood flow play a critical role in regulating the cardiovascular system from early prenatal development until death. Beginning with the first heartbeats, the embryonic primary vascular plexus responds to various hemodynamic forces that modulate plexus differentiation into arteries and veins. Blood flow is a critical requirement for ongoing vascular development, with wall shear stress (WSS) playing a significant role in developmental remodeling of the vascular endothelium. The plasticity of the vasculature in response to prevailing blood flow conditions allows it to rapidly adapt to hemodynamic perturbations, both physiologic and pathologic, with the purpose of maintaining vascular integrity throughout our lives.
Disturbances of blood flow perform an important role in the development of cardiovascular diseases, including atherothrombosis. Chronic disturbances in blood flow lead to vascular changes that promote atherosclerosis, whereas acute changes in blood flow at sites of atherosclerotic plaque disruption are important for the development of thrombosis. Therefore, temporal variations (seconds through to years) in flow are relevant to atherothrombosis. Early seminal work by the German physician, Rudolf Virchow (1856), identified an important role for blood flow disturbances in promoting venous thrombosis. However, an awareness of the central importance of hemodynamics in promoting atherothrombosis did not develop until the 1970s. Two major theories regarding the pathogenesis of atherothrombosis developed at this time: (a) that low and/or oscillatory WSS is important for atherogenesis and (b) that high WSS promotes arterial thrombosis. The purpose of this chapter is to expand on these concepts, highlighting the importance of disturbed “bulk” flow (macroscale flow disturbance) in regulating endothelial cell and platelet function. Furthermore, we focus on an emerging concept in the thrombosis field that localized microscale (1 to 10 µm) changes in blood flow may play a potentially important role in promoting thrombus formation.
ARTERIES RESPOND TO SPECIFIC HEMODYNAMIC FORCES
Blood flow in the arterial circulation is primarily driven by cardiac contractions that create a pressure gradient across the systemic and pulmonary vasculature. A pressure differential pushes blood from regions of high pressure to regions of low pressure, which is resisted by the frictional nature of the vascular wall (Box 29.1). As a result, blood flow creates three dominant stresses within the arterial wall: (a) circumferential stress, (b) axial stress, and (c) WSS, where stress is defined as the distribution of internal forces throughout a deformable body (force/area). Blood vessels are highly responsive to these stresses and modify their overall architecture and composition to maintain a near-constant stress environment that limits metabolic demand and preserves vascular integrity.
Circumferential Stress
Arterial pressure is a major source of stress in the vasculature. Pressure exerts an outward push on blood vessels, creating tension within the arterial wall. Tension results in circumferential stress, which is sensed by smooth muscle cells (SMCs) in the medial layer of arteries. These cells actively oppose this stress to prevent arterial expansion and bursting upon vessel pressurization. Furthermore, elastin and collagen provide additional passive structural support. Average circumferential stress remains at a near-constant value of 105 Pa in the arteries.1 Hypertension creates chronic high pressure increasing circumferential stress and the vessel attempts to alleviate this stress by thickening the medial layer. Such changes to the vessel wall may promote atherosclerosis. The detailed effects of circumferential stress on vascular remodeling have been summarized previously2,3 and will not be discussed further in this chapter.
Axial Stress
A stress that is indirectly linked to hemodynamics is the longitudinal (axial) stress, which exists because arteries are constantly stretched in situ. Axial stress remains near a value of 105 Pa, which is required to avoid vessel buckling (tortuosity) or tearing, and occurs through the modulation of the axial force.3 Both endothelial cells and SMCs can sense axial stress and can signal for control of the vessel microstructure (collagen and elastin composition) in order to modify the internal axial force in response to changes in either the vessel radius or thickness.2 It is interesting to speculate that microstructural changes from axial stress modulation may contribute to plaque formation or degradation, since axial stress is very sensitive to changes in wall thickness, which changes dramatically in the presence of plaque. However, little is known about the role of axial stress in atherothrombosis.
Box 29.1 Hagen-Poiseuille Flow
Blood flow is complex, but the general flow characteristics can be greatly simplified by some basic assumptions, leading to generalized Hagen-Poiseuille flow (see below). An important hemodynamic parameter to blood flow is the shear rate (velocity gradient), which is maximum at the vessel wall and linearly decreases to 0 at the central axis of the vessel for Hagen-Poiseuille flow. The shear stress, t(r), is related to the shear rate by the dynamic viscosity (A). This flow exhibits a parabolic velocity profile, v(r), and is driven by an axial pressure gradient, primarily generated by cardiac contractions (B).
Hagen-Poiseuille Assumptions:
Newtonian fluid—valid for arteries with high shear and high flow—fluid where Newtonian constitutive equation can be applied
Straight, circular pipe far from flow entrance (fully developed flow)
Steady flow
Laminar flow—valid, except in parts of the aorta
There is no slip between fluid and the pipe wall.
Wall Shear Stress
As blood flows through an artery, it creates a pulling force on the endothelial surface, similar to a frictional resistance. The force per unit area applied to and transmitted through the endothelium is equal to the hemodynamic shear stress at the surface (see Box 29.2). WSS is maintained at a near-constant arterial value of 15 to 20 dynes/cm2 through alterations of the luminal radius.1 The purpose, according to Murray’s law, is to optimize the relationship between the flow rate and the arterial radius to reduce the metabolic work required from the body to maintain blood flow.4 WSS is converted into a tensile force within the endothelial cell membrane5 (Box 29.2) and cytoskeleton.
While the contributions of circumferential and longitudinal stresses to atherothrombosis remain poorly defined, the impact of WSS on the atherothrombotic process has been extensively investigated, as described below.
Box 29.2 Wall Shear Stress
WSS, twall, at the fluid-solid interface existing at the luminal side of the endothelium is equal between the shear stress in the blood, twall,blood, and shear stress on the endothelium (A). Therefore, the shear stress experienced by the endothelium can be defined by fluid properties, instead of the endothelial properties. Assuming a fluidic cytosolic interior for endothelial cells, the intracellular shear stress, tintracellular, is negligible and the luminal shear stress is converted into a tension, smembrane, in the cell membrane and on the cytoskeleton (B). In addition to tension and luminal shear stress, there is also a vertical component to shear that resists cell rotation.
THE IMPACT OF WALL SHEAR STRESS ON ATHEROSCLEROSIS
It has long been recognized that certain areas of the vasculature, including the abdominal aorta, carotid bifurcation, coronary, renal, and femoral arteries, are prone to the development of atherosclerotic lesions. These vascular regions are more likely to exhibit chronic inflammatory changes that contribute to intimal thickening.6,7,8,9,10,11 While systemic factors like hypertension, smoking, hyperlipidemia, obesity, and diabetes are associated with atherosclerosis propagation, a key factor determining plaque localization at specific arteries is the underlying regional hemodynamic environment, specifically the presence of low and/or oscillating WSS (FIGURE 29.1). Oscillating WSS refers to the temporal directional transition of WSS during the cardiac cycle, which occurs when near-wall blood flow reverses direction, a
feature of proatherogenic arteries. Localized oscillating WSS promotes atherogenesis through multiple interrelated mechanisms including the tendency for foam cell formation and increased production and retention of proinflammatory mediators. These mechanisms promoting atherosclerosis have been most extensively associated with WSS-dependent altered endothelial structure and function (FIGURE 29.1).
feature of proatherogenic arteries. Localized oscillating WSS promotes atherogenesis through multiple interrelated mechanisms including the tendency for foam cell formation and increased production and retention of proinflammatory mediators. These mechanisms promoting atherosclerosis have been most extensively associated with WSS-dependent altered endothelial structure and function (FIGURE 29.1).
![]() FIGURE 29.1 Regions prone to plaque formation include vessels with branches and sharp curvatures, corresponding to regions of altered velocity profiles. Areas of low and/or oscillating WSS can develop in these situations (light gray regions), which may promote atherogenesis. This process is promoted through numerous pathways, including endothelial cell morphologic and functional changes. One structural change is the altered shape and misalignment of neighboring ECs in regions of low and/or oscillating WSS (arrows representing WSS over ECs), resulting in increased endothelial permeability. Furthermore, these structural changes are associated with regions of chronic inflammation. Intimal thickening occurs most rapidly in regions of low and/or oscillating WSS, as determined by intravascular ultrasound.11,12,13 Plaques found in these regions are commonly expansive, extending in the abluminal direction and preserving the luminal area. Expansive plaque generally has vulnerable characteristics, including a large lipid core, inflammatory infiltrate, and a thin fibrous cap.14,15 Therefore, vessel geometries are closely tied in with atherosclerosis through the resulting effects on WSS distribution. |
Impact of Wall Shear Stress on Endothelial Cell Function
Endothelial cells play a key role in maintaining vascular integrity and in dynamically regulating vascular function. This occurs through several mechanisms including the regulation of vascular permeability, remodeling, synthesis, and secretion of vasoactive, prothrombotic, and antithrombotic molecules, as well as through the modulation of vascular tone through interactions with SMCs. The principle hemodynamic driver of endothelial cell function is the applied WSS. The response of endothelial cells to the WSS environment is complex and wide ranging, with changes in WSS triggering intracellular signaling pathways (FIGURE 29.2A) that modulate gene expression and cell morphology.12
Endothelial cells “sense” changes in blood flow, specifically oscillatory WSS (FIGURE 29.2A), and convert these mechanical signals into chemical signals (mechanotransduction) that modulate endothelial cell structure and function (FIGURE 29.2B). The luminal surface of the vascular endothelium contains several key membrane structures that are considered to be important for their mechanosensory function13,14,15,16:
Caveolae—these are small surface invaginations of the endothelial cell plasma membrane linked to the initiation and propagation of Ca2+ signaling in response to WSS. These functional lipid membrane compartments are important for shear dependent up-regulation of nitric oxide (NO) synthesis and represent a primary membrane component of the mechanosensory machinery.
Primary cilia—these are extensions of the endothelial cell plasma membrane that undergo mechanical deformation in response to blood flow. Bending of primary cilia is thought to lead to the opening of mechanically gated ion channels leading to Ca2+ influx and endothelial cell activation.
The glycocalyx—this is comprised of a network of glycosylated and sialylated transmembrane proteins protruding from the plasma membrane into the vessel lumen and has been found to directly respond to applied mechanical shear forces. Under low-flow conditions, this glycoprotein network exists in a tightly coiled state. With the application of blood flow, the glycocalyx uncoils in the direction of flow, leading to transmembrane conformational signaling. Significantly,
the glycocalyx serves to regulate vascular permeability, with increased permeability at oscillatory WSS resulting from an overall reduction in glycocalyx thickness.
![]() FIGURE 29.2 Endothelial cell responses at low and/or oscillatory WSS. A: Oscillatory WSS occurs in vascular regions where the near-wall flow changes direction during the cardiac cycle. These regions also typically correspond to regions of low WSS. When the near-wall flow changes directions, the overall WSS and drag force on the EC changes directions. Low/oscillatory WSS can occur even in regions where the bulk flow generally remains in monodirectional. These situations are possible in poststenotic regions and in the regions of a sinus, where the vessel expands abruptly. B: The luminal surface of the EC plasma membrane directly interfaces with blood flow and represents the sensing surface of the endothelium. On this surface are multiple mechanosensing elements, the caveolae, glycocalyx, and primary cilia. C: Multiple EC responses occur as a result of low/oscillatory WSS signaling, creating a proatherogenic environment. The extracellular matrix (ECM) is degraded by MMPs, while synthesis is down-regulated. SMC migration, differentiation, and proliferation are increased. The balance of vasomodulation is weighted toward vasoconstriction. Also, the overall EC morphology and alignment are disrupted, such that there are larger gaps between ECs. Figure is modified by permission from Chatzisisis et al.23 |
In addition to mechanosensors on the luminal surface of endothelial cells, mechanical signals can also be transduced from the abluminal surface of endothelial cells, with integrin receptors on the basal plasma membrane playing a major role in transducing signals to the nucleus through the actin cytoskeleton (FIGURE 29.2B). The details underlying integrin mechanotransduction have been investigated in considerable detail and reviewed elsewhere17 and will not be discussed further here.
Atheroprotective Signals
Endothelial mechanosensors generally produce atheroprotective signals when the flow is unidirectional, laminar, and at a WSS that is >10 dynes/cm2. A key antiatherogenic effect of endothelial cells is the up-regulated synthesis of NO and prostacyclin (PGI2), two major vasodilators that regulate local vascular tone and profoundly inhibit platelet activation and leukocyte adhesion.18,19,20 Furthermore, such flow conditions also suppress endothelial cell proliferation and promote cell survival, through up-regulated expression of antiapoptotic genes.21,22 In combination, these cumulative effects help reduce the proinflammatory and prothrombotic phenotype of endothelial cells, thereby inhibiting the development of atherosclerotic lesions.
Proatherogenic Signals
Atherosclerosis is promoted by localized disturbances in blood flow, specifically low oscillatory WSS (FIGURE 29.2A) and
temporal shear gradients (Box 29.3).23,24,25,26 Spatial WSS gradients (Box 29.4) may also promote atherogenesis, although the absolute importance of these flow changes to the atherosclerotic process remains unclear.27,28,29 The effects of shear gradients on endothelial function and structure are complex and wide ranging. For example, temporal shear gradients and low oscillatory WSS have been demonstrated to activate the MAPK23,25,30 and PI 3-kinase signaling pathways (FIGURE 29.2B) leading to activation of the nuclear shear-sensitive response elements and the induction of proatherogenic transcription factors.23,25 As a consequence, there is increased expression of ICAM-1 and E-selectin on the surface of endothelial cells23,26 and increased production of the chemokine MCP-1.23,24 These changes enhance monocyte recruitment. Local disturbances in blood flow also promote the synthesis of growth factors, such as PDGF-A, which has proatherogenic and proangiogenic properties (FIGURE 29.2C).24,31
temporal shear gradients (Box 29.3).23,24,25,26 Spatial WSS gradients (Box 29.4) may also promote atherogenesis, although the absolute importance of these flow changes to the atherosclerotic process remains unclear.27,28,29 The effects of shear gradients on endothelial function and structure are complex and wide ranging. For example, temporal shear gradients and low oscillatory WSS have been demonstrated to activate the MAPK23,25,30 and PI 3-kinase signaling pathways (FIGURE 29.2B) leading to activation of the nuclear shear-sensitive response elements and the induction of proatherogenic transcription factors.23,25 As a consequence, there is increased expression of ICAM-1 and E-selectin on the surface of endothelial cells23,26 and increased production of the chemokine MCP-1.23,24 These changes enhance monocyte recruitment. Local disturbances in blood flow also promote the synthesis of growth factors, such as PDGF-A, which has proatherogenic and proangiogenic properties (FIGURE 29.2C).24,31
Box 29.3 Temporal Wall Shear Stress Gradients
The cardiac cycle causes pulsatility in major arteries, including the coronary, carotid, femoral, and aortic arteries. Therefore, there is almost always a low temporal gradient in WSS for these arteries. Diseased arteries, specifically those with a stenosis, exhibit much larger oscillations in flow with respect to time, creating larger gradients in WSS.39,40 In terms of cells in free flow (e.g., platelets) and cells at a vessel surface (e.g., ECs), both experience changes in WSS due to the pulsatility.
Flow rates through major atherosclerotic-prone arteries oscillate during the cardiac cycle. The waveform shown in this figure is an arbitrary waveform that has some characteristic features of flow through these arteries. Actual flow rate waveforms differ from artery to artery. During systole, flow rates accelerate (i) in most arteries, as pressure builds up in the aortic arch. Flow rates become the highest right before diastole (ii). During diastole (iii), the flow decelerates in most arteries. In the coronary arteries, the converse is true. During this cycle, flow can reverse direction (iv) in the coronary, femoral, and carotid arteries. Oscillatory WSS occurs when near wall blood reverses direction, such as the region consisting of sections (iii) and (iv).
WSS generally varies in a similar way as the flow rate, as illustrated by the proportionality between the two for Hagen-Poiseuille flow. However, it also depends on the velocity waveform, which changes throughout the cardiac cycle. The WSS presented here is an idealized case that is based solely on the flow rate and is independent of the velocity waveform, for the purpose of clarity.
A temporal WSS gradient exists at a given location because of blood pulsatility. The gradient depends on local arterial conditions. It is maximum during flow acceleration and is minimum during flow deceleration. The temporal WSS gradient is the slope of the WSS versus time curve.
The pulsatility of blood flow creates an unsteady flow field. Velocity profiles do not remain parabolic, but instead can be characterized by the Womersley solution.10,41,42 Velocity profiles are a commonly used method to visualize fluid mechanics but can be conceptualized as the amount a fluid moves for a given time and how that varies across the diameter of the blood vessel. The fluid velocity can partially (iii -not shown in B) or completely (iv) change directions during the cardiac cycle, depending on the artery and geometrical conditions, corresponding to the roman numerals over the flow waveform plot in (A).Stay updated, free articles. Join our Telegram channel
Full access? Get Clinical Tree
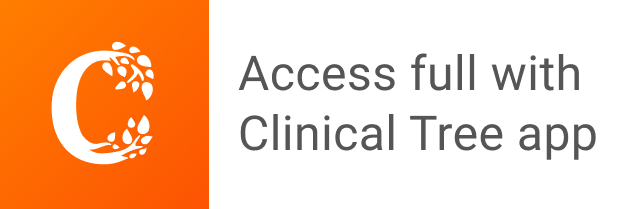