Retinopathy
Jing Chen
Andreas Stahl
Lois E. H. Smith
The eye is one of the most important sensory organs that enables us to perceive the environment to ensure species survival. The light-sensitive part of the eye, the retina, is composed of several transparent neuronal layers and functions similarly to a film in a camera. Light focused through the cornea and lens passes across the transparent retinal layers to interact with light-sensitive pigments in the photoreceptor outer segments, causing a signal transduction cascade. The signal generated in the photoreceptor ultimately propagates a series of action potentials across the retina, through the optic nerve to the neurons in the visual cortex of the brain, and results in the perception of an image (FIGURE 74.1). The essential function of the retina in light perception and processing is dependent upon a well-organized nonleaky vascular network that supplies vital oxygen and nutrients and clears waste. In retinal vascular diseases, the normal function of the ocular vasculature is disrupted, often leading to loss of barrier function with resulting plasma exudation and hemorrhage and, in most severe cases, disturbance of retinal neuronal function and loss of vision.
Epidemiologic studies have shown that proliferative diabetic retinopathy (PDR) accounts for the highest incidence of acquired blindness in the working-age population1,2; choroidal (subretinal) neovascularization in age-related macular degeneration (AMD) represents the leading cause of blindness in people over the age of 653,4; and retinopathy of prematurity (ROP) is a major cause of acquired blindness in children5 (FIGURE 74.2). In addition, there are many other ocular diseases that have a vascular component, such as retinal vein occlusion (RVO), Norrie disease, familial exudative vitreoretinopathy (FEVR), Coats disease, sickle cell retinopathy, retinitis pigmentosa, and others. This chapter summarizes our current knowledge of the physiology of the ocular vasculature and pathophysiology of several common vascular eye diseases, as well as cellular and molecular mechanisms that regulate the growth of ocular vessels.
OCULAR VASCULATURE IS COMPOSED OF THREE SEPARATE VASCULAR BEDS: HYALOIDAL, CHOROIDAL/UVEAL, AND RETINAL VESSELS
Hyaloid Vasculature
The hyaloid vasculature is a transient embryonic vascular bed that develops during embryonic and fetal stages to provide blood supply to the developing eye. The hyaloid vascular plexus originates from the optic nerve, extends through the primitive vitreous, and surrounds the developing lens. The hyaloid vessels eventually regress with completion of eye development, usually before 34 weeks of gestation in humans. In rare diseases such as Norrie disease and FEVR, the hyaloid vasculature fails to regress, resulting in persistent fetal vasculature, which is associated with poorly developed neural retina and impairment of vision.
Choroidal Vasculature
The choroidal vasculature is part of the uveal system of vessels that perfuse the iris, ciliary body, and choroid (FIGURE 74.1). During optic cup development, the primitive choroidal vascular system is formed from the surrounding mesoderm. Later, following the development of retinal pigment epithelium (RPE), this primitive vascular layer expands further,6 until the capillaries completely encircle the optic cup. A definitive choriocapillaris layer appears at around 8 to 12 weeks of gestational age.
The choroidal vasculature consists of larger posterior vessels and a small-caliber anterior choriocapillaris (FIGURE 74.2), adjacent to the RPE. The choriocapillaris is a sinusoidal vascular plexus and is the site of the greatest blood flow (65% to 85%) in the eye.7 The choriocapillaris provides oxygen and nutrients to the outer retina, particularly the RPE and photoreceptors that have the highest metabolic rate in the body and therefore have a very high oxygen demand. The choriocapillaris is highly fenestrated and allows blood flow beneath the basal surface of the RPE that digests shed photoreceptor outer segments and clears other photoreceptor waste. The RPE with tight junctions on the apical surface adjacent to the photoreceptors constitutes the outer blood retinal barrier (BRB). Similar to the blood brain barrier, the BRB is vital to preserve tissue integrity and function of the retina. The inner BRB is formed by vascular endothelial cells of the inner retina with tight junctions. The endothelial cells abut Müller cell endfeet and pericytes. This inner BRB is compromised in diabetic retinopathy, leading to retinal edema and often severe visual impairment. In exudative AMD in contrast, it is the outer BRB that is compromised when choroidal blood vessels (without tight junctions) grow through Bruch membrane and into the subretinal space, breaking through the RPE’s BRB. This leads to edema of the macula, the part of the retina that is responsible for central vision and higher visual functions like reading or recognizing faces.
Retinal Vasculature
In the eye, 20% to 30% of blood flow is supplied by the retinal circulation, serving mainly the inner retina. In humans, retinal vascularization starts in utero at about 16 weeks of gestational age and is completed at approximately 40 weeks of gestation, right before birth. The retinal vessels develop as the hyaloid vessels regress. During development, retinal vessels grow first in the most superficial (i.e., innermost) layers of the retina and
sprout radially from the optic nerve toward the ora serrata, the peripheral edge of the retina. They reach the nasal side of the ora serrata at about 36 weeks of gestational age, and the temporal retina at approximately 40 weeks of gestational age. As the superficial layer is almost completed, retinal vessels dive into the retina to form first a deep and then an intermediate layer along with a well-organized network of interconnecting vessels to complete three vascular layers: a superficial vascular layer that lies in the inner part of the nerve fiber layer, an intermediate layer in the inner plexiform layer, and a deep layer in the outer plexiform layer.8 Similar to the RPE, the retinal vessels maintain BRB integrity.9
sprout radially from the optic nerve toward the ora serrata, the peripheral edge of the retina. They reach the nasal side of the ora serrata at about 36 weeks of gestational age, and the temporal retina at approximately 40 weeks of gestational age. As the superficial layer is almost completed, retinal vessels dive into the retina to form first a deep and then an intermediate layer along with a well-organized network of interconnecting vessels to complete three vascular layers: a superficial vascular layer that lies in the inner part of the nerve fiber layer, an intermediate layer in the inner plexiform layer, and a deep layer in the outer plexiform layer.8 Similar to the RPE, the retinal vessels maintain BRB integrity.9
![]() FIGURE 74.1 Schematic illustration of (A) normal eye and (B) proliferative retinopathy with pathologic neovascularization. |
Under pathologic conditions when the BRB is disrupted, the retinal vessels become more permeable resulting in retinal edema and vision loss. This is seen with ROP and diabetic retinopathy, when retinal vessels proliferate to form fragile leaky neovessels. RVO is also a common retinal vascular disease. In the early stages of RVO, plasma leakage and hemorrhage is due to increased intraluminal pressure in the obstructed vein; in later stages it is due to vascular endothelial growth factor (VEGF)-induced proliferation of leaky neovessels and VEGF-induced increased permeability of normal vessels.
![]() FIGURE 74.2 Vascular cast of choroidal vasculature taken with a scanning electron microscope. Scale bar: 200 µm. Image courtesy of Dr. Przemyslaw Sapieha, University of Montreal, Montreal, Canada. |
Among these three blood vessel systems in the eye, the inner retinal vasculature is the most extensively studied. The retina is an excellent model of vascular development, vessel remodeling, and vascular maturation. Studies of retinal vasculature have greatly expanded our basic understanding of the factors that regulate normal and pathologic vascularization including the relationship between oxygen and retinal vascular growth.
OXYGEN REGULATES BLOOD VESSEL GROWTH IN THE EYE
The hypothesis that oxygen-sensitive molecules control the development of retinal vasculature originates from early observations that capillaries grow more profusely near venules carrying oxygen-depleted blood than around arteries with fully oxygenated blood.10,11,12 The vascular pattern of some eye diseases also supports the idea that oxygen (or lack of it) regulates blood vessel growth in the retina. Pathologic retinal angiogenesis in ROP and diabetic retinopathy is preceded by an initial loss of retinal vessels with resulting retinal ischemia, suggesting that hypoxia is an important stimulator of new blood vessel growth.13 The timing and spatial pattern of normal retinal vascularization is coordinated with the differentiation, maturation and increasing metabolic demand of neural and glial cells. The wave of maturation of neural retina creates a moving wave of “physiologic hypoxia” that guides the development of new vasculature from the center toward the periphery of the retina.14 VEGF, an essential growth factor for vascular growth and
survival is induced in a pattern that coincides with retinal blood vessel development both temporally and spatially.15,16,17 As new vessels form, bringing oxygen and suppressing VEGF, the wave moves forward toward the developing retina.
survival is induced in a pattern that coincides with retinal blood vessel development both temporally and spatially.15,16,17 As new vessels form, bringing oxygen and suppressing VEGF, the wave moves forward toward the developing retina.
Retinal Tissue Senses Oxygen Tension through Hypoxia-Inducible Factor
The increased expression of VEGF and some other blood vessel growth factors in response to low tissue oxygen levels is mediated in large part through the transcription factor hypoxia-inducible factor 1 (HIF1), which functions as a global regulator of O2 homeostasis.18,19,20 HIF1 is a heterodimeric protein composed of an oxygen-sensing α unit and a constitutively expressed transcription activating β subunit. Under normoxic conditions, a proline residue in HIF1α is hydroxylated by Egl9 enzyme, facilitating the interaction between HIF1α and von Hippel-Lindau protein, resulting in the ubiquitylation and proteasomal degradation of HIF1α.21,22,23 Under hypoxic conditions, HIF1α escapes prolyl hydroxylation and dimerizes with HIF1β. The HIF1 heterodimer then translocates to the nucleus and activates target genes by binding to a hypoxia responsive element in the promoter region.18 In addition to VEGF, erythropoietin (Epo) is an important target gene of HIF1 that plays an important role during retinal blood vessel homeostasis.24,25,26 Epo is a glycoprotein hormone that is best known for its stimulation of erythropoiesis. However, Epo has also potent neuroprotective and proangiogenic effects. In the vitreous of patients with diabetic retinopathy,26,27 ROP28 or RVO,29 Epo is upregulated along with VEGF, which is one of the master regulators of both normal retinal vessel development and proliferative retinopathies.15,30,31
VEGF Guides Retinal Vascularization Following an Astrocytic and Neuronal Template
Although tissue oxygen tension is an essential stimulus for retinal vessel growth, hypoxia alone does not generate the organized pattern of retinal vascularization and the structural alignment among retinal vessels, astrocytes and neurons that is seen during normal retinal development. It has been suggested that several other cellular guides contribute to this highly organized pattern of retinal vessel growth. Astrocytes grow along the inner surface of the retina preceding the vessels. It is assumed that this astrocytic template secretes VEGF and other factors in a tightly controlled manner to create a highly specialized micro milieu to guide the filopodia of sprouting endothelial cells in the right direction.8,32,33,34 It is also postulated that the relative hypoxia of the deeper retinal layers during development results in a VEGF gradient that favors sprouting from the superficial layer downward, resulting in the formation of first the deep and then the intermediate layers of capillary networks.15,16 If the astrocytic template guides vascular growth in the retina, what is it that guides the formation of the astrocyte template during retinal development? One theory postulates that astrocyte growth follows the radially oriented ganglion cell axons,8,33 which synthesize platelet-derived growth factor (PDGF) to stimulate astrocyte growth and alignment.35 The radial cellular pattern in the retina is likely to result from complex and highly regulated reciprocal cellular interactions: retinal neurons serve as the primary template and secrete PDGF to stimulate astrocyte growth and patterning; astrocytes in turn respond to increasing hypoxia during retinal maturation by increasing the synthesis and secretion of VEGF to induce and sculpt the formation of a retinal vascular plexus. When the vascular plexus becomes functional, it alleviates tissue hypoxia, decreasing VEGF expression and limits further excessive vascular growth.36,37
Remodeling of Retinal Vasculature Is Also Dependent on Oxygen
Once formed, the superficial layer of retinal vessels is remodeled before sprouting to the deeper layer,38 which is regulated by the specific needs of the local tissue for oxygen and nutrients. Maturation of the superficial plexus leads to a less dense network with finer vessels compared to the structures formed during early development. Relative hyperoxia around arteries is considered an important factor for pruning of existing vasculature. Local hyperoxia would lead to local suppression of VEGF, which is an endothelial survival factor for immature capillaries. Reduction of VEGF leads to regression of these immature capillaries in areas with excess vasculature.39,40 Leukocytes from the circulation also participate in vessel remodeling through induction of endothelial cell apoptosis, a process considered most relevant in the context of early vessel loss in diabetic retinopathy.38
VEGF during Development of the Choroidal Vasculature
Choroidal vascular development is less well studied than the development of the retinal vasculature. Clinically the retinal vasculature can be readily observed funduscopically in humans, while the choroidal plexus is blocked from view by a dense layer of pigmented RPE cells. Furthermore, in animal models, retinal vasculature development can readily be studied due to the postnatal development of retinal vessels in rodents. Retinal flat mounts expose retinal vessels well. The choroidal vasculature, in contrast, is less readily quantifiable during development. Nevertheless we know that formation and maturation of the choroidal vasculature is tightly associated with signals produced by RPE cells.41 In particular, VEGF is highly expressed by RPE cells at the time of choriocapillaris formation,42,43 suggesting involvement of RPE-derived VEGF in the development of choroidal vessels. Furthermore, in colobomas, failure of RPE differentiation results in defective development of the choroid and sclera,44 and in patients with exudative AMD, degeneration of RPE cells and associated overproduction of VEGF leads to pathologic activation and sprouting of the normally quiescent choriocapillaries.
VEGF and Wnt Signaling during Formation and Regression of the Hyaloid Vasculature
The hyaloid artery penetrates into the optic cup in early development and extends through the vitreous to form branches that envelop the forming lens. Retinal vasculature development coincides with hyaloid vessel regression.6,45 The mechanism governing both formation and regression of hyaloid vessels is still incompletely defined. VEGF was suggested to trigger the growth of hyaloid vessels because VEGF is expressed in the portion of the lens closest to the forming vessels.42,46,47 In addition, patients
with VEGF-induced endothelial cell hyperplasia also have disorganized and persistent hyaloid vessels, further supporting a role of VEGF in hyaloid vessel formation.48
with VEGF-induced endothelial cell hyperplasia also have disorganized and persistent hyaloid vessels, further supporting a role of VEGF in hyaloid vessel formation.48
Interestingly, an entirely different process has been uncovered for hyaloid vessel regression (and retinal vascular formation) from studies investigating a group of rare hereditary eye diseases, Norrie disease, and FEVR. These diseases have in common persistent hyaloid vessels and incomplete formation of the retinal vasculature with a lack of a deep retinal plexus formation.49 They arise from a number of mutations in the canonical Wnt-β-catenin signaling pathway: Wnt ligands Norrin or Wnt receptors Frizzled4 and Lrp5 are involved and mediated in part through macrophages.50,51
OCULAR DISEASES WITH VASCULAR PATHOLOGY
Many retinal eye diseases result from disruption of the normal blood supply. The subsequent hypoxia often triggers pathologic neovascularization. In diabetic retinopathy and ROP, abnormal proliferating retinal vessels extend into the vitreous. In AMD, choroidal vessels can proliferate pathologically into the normally avascular subretinal space. RVO is another common eye vascular disease, which in its late stages can lead to pathologic retinal vessel proliferation. The following sections discuss the clinical features of these common ocular vascular diseases and our current understanding of the pathogenesis and treatment options.
Retinopathy of Prematurity
ROP is a major cause of blindness in children in the developing and developed world despite current surgical and laser treatment in the late stage of the disease.52 Formerly known as retrolental fibroplasia, ROP was originally described in the 1940s when the condition was first connected with oxygen supplementation after premature birth.53 Initially, no treatment was available. Major advances came in the 1980s, when cryoablation of avascular retinal areas was shown to be partially effective in preventing blindness in ROP infants.54 Laser photocoagulation soon supplanted cryotherapy.55 However, although ablation treatment can reduce the incidence of blindness by approximately 25% in infants with late-stage disease, it does not improve the chances of good visual acuity. Preventive and less destructive therapies for ROP are thus highly desirable.
Risk Factors of ROP
ROP was associated with excessive oxygen use shortly after the initial description of the disease.56,57 Controlled supplemental oxygen is now delivered to premature infants to maintain adequate blood levels of oxygen but at the same time limit the development of ROP.58 However, even with monitored oxygen use, the incidence of ROP has increased over the last decades, probably due to the increased survival of infants with very low birth weight.59 Oxygen use, early gestational age and low birth weight are major risk factors for ROP.60,61,62 However, the postnatal factors of low postnatal weight gain, as well as low insulin-like growth factor (IGF)-1 blood levels are as important as gestational age and birth weight in determining ROP progression, as well as increased blood glucose level, which is also recognized as a risk factor.63,64,65,66,67,68,69
Pathogenesis of ROP: Two Phases
ROP is a biphasic disease consisting of an initial phase of impaired retinal vessel growth followed by a second phase of vascular repair that is—in severe cases—accompanied by pathologic vessel proliferation in the retina and often into the vitreous. Infants born prematurely have an incompletely vascularized retina at birth. During the first phase of ROP, the peripheral avascular zone of premature infants becomes vascularized too slowly to keep up with the rising metabolic demand of the maturing neurosensory tissue. The severity of ROP is to a large extent determined by the size of avascular retina (FIGURE 74.3), which in turn is dependent on gestational age at birth. The earlier an infant is born, the less vascularization of the retina has occurred. In addition to prematurity, a hyperoxic environment relative to that found in utero and additional oxygen treatment, which is often needed for survival of prematurely born infants, contributes to the slowing (or even cessation) of normal vascular growth. In utero, the normal PaO2 is 30 mm Hg, compared to a PaO2 of 60 to 100 mm Hg of an infant breathing room air.70 As a consequence, in utero the blood is only approximately 70% saturated with oxygen compared to 100% in infants in room air. This first phase of impaired retinal vascularization signifies the first phase of ROP, which spans from birth to about 30 to 32 weeks of gestational age.
During the second phase of ROP, the retina changes from hyperoxic to hypoxic. It is believed that this change is induced by increased metabolic demand of the maturing neurosensory retina. During this phase neuronal and glial cells in the avascular areas of the peripheral retina are oxygen and nutrient deficient. This local tissue hypoxia leads to upregulation of proangiogenic growth factors that induce the ingrowth of new blood vessels. This second phase of ROP, characterized by hypoxia-triggered increased blood vessel growth, begins around 32 to 34 weeks of gestational age. However, in severe cases of ROP, with large avascular areas, the upregulation of angiogenic factors is excessive and induces not only organized growth of functional vessels but also uncontrolled sprouting of pathologic vessels in the retina and from the retina proper into the normally avascular vitreous. These preretinal neovessel proliferations are very similar to the pathologic neovascularization seen in other proliferative
retinopathies such as diabetic retinopathy or the late stages of RVO. Several severe complications can arise from these pathologic neovessels: first, the preretinal nature of some of these neovessels exert tractional forces leading to detachment of the retina from the underlying RPE; second, the unorganized structure of these neovessels leads to plasma leakage and bleeding into retina and/or vitreous. These complications can lead to blindness or severe visual impairment. Even with treatment, patients who progress to these severe stages of ROP have usually extremely limited chances of developing normal or near-normal visual function.
retinopathies such as diabetic retinopathy or the late stages of RVO. Several severe complications can arise from these pathologic neovessels: first, the preretinal nature of some of these neovessels exert tractional forces leading to detachment of the retina from the underlying RPE; second, the unorganized structure of these neovessels leads to plasma leakage and bleeding into retina and/or vitreous. These complications can lead to blindness or severe visual impairment. Even with treatment, patients who progress to these severe stages of ROP have usually extremely limited chances of developing normal or near-normal visual function.
VEGF in ROP
VEGF is an oxygen-regulated factor essential for normal retinal vascular development and important in ROP. As one of the most potent proangiogenic molecules,71,72,73 VEGF can be secreted by a wide variety of cell types in hypoxic conditions.74,75,76 Elevated VEGF levels are found in the vitreous of patients with retinal neovascularization,75,77 including those with ROP.78 In the neovascular second phase, antiangiogenic treatment using an anti-VEGF aptamer or anti-VEGF antibodies or antibody fragments might be beneficial. However, although anti-VEGF therapies have produced beneficial results in patients with neovascular AMD, the efficacy and safety of anti-VEGF treatments in ROP have still to be determined. As a consequence, anti-VEGF therapy in the second stage of ROP cannot be recommended at the time of writing as too little data exists on potentially severe side effects of anti-VEGF treatment in premature infants.
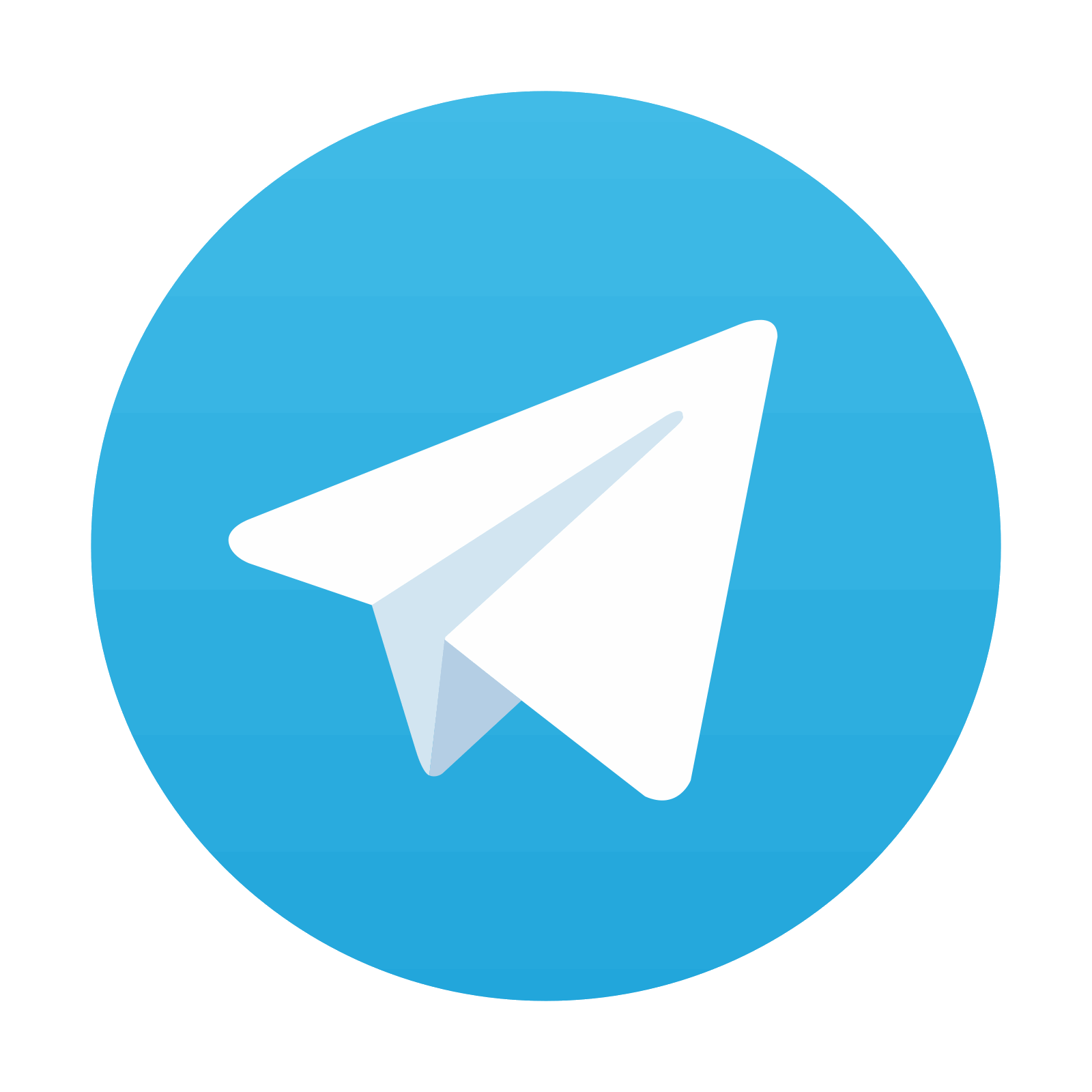
Stay updated, free articles. Join our Telegram channel
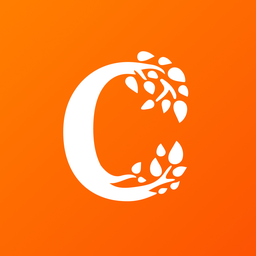
Full access? Get Clinical Tree
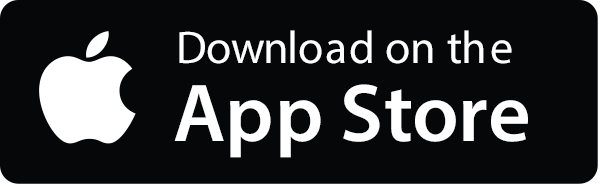
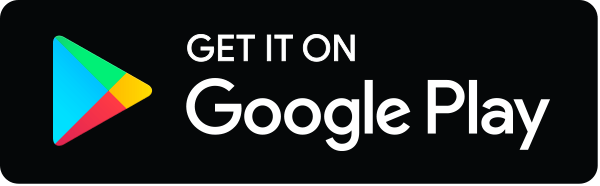
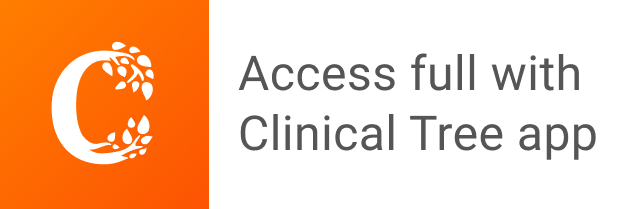