In the modern age of cancer therapy, advances in the multidisciplinary management of cancer have resulted in increased rates of survivorship. Radiation therapy (RT) toxicity must be tempered with the desire to achieve dose escalation to provide the best chance of long-term cure. This article is designed to acquaint the reader with common, expected, and potential acute and late complications of RT.
In the modern age of cancer therapy, multidisciplinary management has led to increasing rates of survivorship. Surgery, chemotherapy, and radiation therapy (RT) are often integrated to optimize the likelihood of local control and survival for a wide spectrum of malignancies. RT toxicity must be tempered with the desire to deliver intensive therapy with the goal of improving tumor control and cure. The differential diagnosis for many RT-related toxicities is extensive. Several factors can modulate RT-related toxicity, including ancillary treatments, such as surgery and chemotherapy, as well as the underlying nutritional and medical status of the patient. This article is designed to acquaint emergency medicine physicians with common toxic effects in patients undergoing RT.
Therapeutic mechanism of clinical radiation therapy
The principal therapeutic beam in radiation oncology is the x-ray (or γ-ray), and it consists of a large number of photons, or “packets,” of energy. The absorption of energy from radiation in a biologic material may lead to excitation or to ionization within the atoms of the biologic tissue. The raising of an electron in an atom or molecule to a higher energy level without actual ejection of the electron is called excitation. If the photon is of sufficient energy, ejection of 1 or more orbital electrons from the atom can occur, and this process is referred to as ionization. The radiation that causes this is referred to as ionizing radiation. The most important characteristic of ionizing radiation is the localized release of large amounts of energy. The energy dissipated per ionizing event (33 eV) is more than enough to break strong chemical bonds. Charged particles, such as electrons, protons, and α-particles, are directly ionizing, whereas uncharged particles, such as photons and neutrons, are indirectly ionizing. Provided that charged particles have sufficient kinetic energy, they can directly disrupt the atomic structure of the biologic material through which they pass, creating chemical and biologic damage. Uncharged particles, with energy similar to photons, do not directly produce chemical and biologic damage themselves, but when they are absorbed in the material through which they pass, they give up their energy to produce fast-moving charged particles, known as free radicals, that are able to produce damage.
Conventional RT involves using x-rays to produce biologic damage. When a photon hits a water molecule in a cell, generation of a hydroxyl free radical occurs. It is estimated that two-thirds of the x-ray damage to DNA in mammalian cells is caused by the hydroxyl radical. Damage to cellular DNA is the primary mechanism through which radiation is able to exert its therapeutic effect. When a cancer attempts to divide with a damaged genome, it undergoes a mitotic death.
Radiobiological effects of radiation therapy at the cellular and tissue level
RT is a local modality that generally causes toxicity limited to the area of treatment (with some caveats). The effects of RT on normal tissues generally result from the depletion of a cell population by cell killing. The cells of normal tissue are not independent units but rather form a complete integrated structure. Response to RT is governed by 3 main factors: (1) inherent cellular radiosensitivity, (2) kinetics of the tissue, and (3) organization of cells within the tissue. Visible damage to an organ becomes evident only if a sufficient proportion of cells are killed. As mentioned previously, cell death after irradiation occurs when cells try to divide. In tissues with rapid turnover, damage can become evident quickly—in a matter of hours (as in gastrointestinal [GI] mucosa) or days (skin). This gives rise to the concept of “early” responding tissues. “Late” responding tissues have toxicity that occurs after a delay of months or years and predominantly in slowly proliferating tissues, such as the lung, kidney, heart, liver, and the central nervous system (CNS). Acute damage is generally repaired quickly secondary to rapid proliferation of underlying stem cells. Late effects, in contrast, may improve but never completely repair. A late effect generally results from a combination of vascular damage through radiation endarteritis in combination with a loss of parenchymal cells. Radiation endarteritis leads to significant atherosclerotic disease several years after initial therapy, in the areas that previously received RT. If an intensive radiation regimen is used with subsequent depletion of the stem-cell population below levels needed for tissue repair, an early reaction in a rapidly proliferating tissue may persist as a chronic injury. This is termed a consequential late effect. An example of this would be necrosis or fibrosis of the skin that occur consequent to severe radiation dermatitis and acute ulceration.
Another concept that is important in understanding radiation toxicity is the idea of an underlying organ’s makeup, namely its functional subunits. The tolerance of normal tissues to radiation is dependent on the ability of clonogenic tissue progenitor cells to maintain a sufficient number of differentiated cells that are appropriately structured to maintain organ function. The structural organization of a tissue is crucial in determining the relationship between survival of clonogenic progenitor cells and organ function versus failure. Structurally defined functional subunits can include the hepatic lobule, the renal nephron, or the pulmonary acinus. Structurally undefined functional units are seen in the cutaneous skin where re-epithelialization of a denuded area can occur either from surviving clonogens within the affected area or by migration from adjacent areas. In the case of organs such as the lung, liver, and kidney, a certain portion can be treated without significant dysfunction. The subunits in these tissues work in parallel to carry out the physiologic function. This is in contrast to serial tissues, such as the spinal cord, where disruption of a portion of the tissue affects all downstream activity.
RT is generally given as a number of small doses on a daily basis, rather than one large fraction (although this does occur as well and is generally referred to as radiosurgery). Dividing a dose into a number of smaller doses, or fractions, allows normal tissues to be spared. This is due to repair of cellular damage that occurs each day. Normal cells typically have better repair mechanisms than those of cancer cells, and RT is used to exploit this difference. Prolonging overall treatment time and decreasing fraction size will generally decrease the severity of “early” effects. Increasing the dose of radiation per fraction to normal tissue may not affect the patient acutely but results in an increased risk of “late” effects several months to several years later. A review of classically defined radiation tolerance is shown in Table 1 . The TD 5/5 and TD 50/5 in Table 1 refer to radiation doses that would be expected to cause a 5% or 50% rate of the given complication or toxicity at 5 years.
Organ | TD 5/5 (Dose in Gy) | TD 50/5 (Dose in Gy) | Clinical Endpoint | Management Considerations |
---|---|---|---|---|
Kidneys | 23–50 | 28–40 | Nephritis | Expectant/dialysis |
Bladder | 65–80 | 80–85 | Bladder contracture/volume loss/refractory hematuria | Consider hyperbaric oxygen (HBO) |
Bladder | Acute cystitis/spasm | Analgesics (eg, phenazopyridine) and/or antispasmodics (eg, oxybutynin) | ||
Femoral head | 52 | 65 | Necrosis | —— |
Skin | 55–70 | 70 | Necrosis, ulceration | Wound/burn management (silvadene, antibiotics, skin grafting if severe) |
Skin | Acute desquamation | Moisturizing creams, hydrocortisone cream, hydrogel wound dressing, consider empiric antibiotics | ||
Bone (mandible) | Osteoradionecrosis | Surgical debridement, antibiotics, consider HBO | ||
Oral mucosa | 60 | 75 | Ulceration, fibrosis, mucositis | Wound care, oral hygiene and baking soda mouthwash, gelclair, narcotics, topical anesthetics, consider empiric antifungal |
Brain | 45–60 | 60–75 | Necrosis, infarction | Corticosteroids, mannitol, neurosurgical evaluation |
Spinal cord | 47–50 | 70 | Myelitis, necrosis |
|
Brachial plexus | 60–62 | 75–77 | Plexopathy, nerve damage | HBO questionable, high-dose steroids questionable, physical therapy |
External/middle ear | 30–55 | 40–65 | Acute/chronic serous otitis | Earwax removal (Debrox), pseudoephedrine, myringotomy in severe cases |
Parotid | 32 | 46 | Xerostomia | Pilocarpine, artificial saliva, antibacterial mouthwash, dental gum |
Lung | 18–45 | 25–65 | Pneumonitis | Corticosteroids, antibiotics |
Heart | 40–60 | 50–70 | Pericarditis | Corticosteroids, nonsteroidal anti-inflammatory |
Esophagus | 55–60 | 68–72 | Stricture, perforation | Dilation |
Esophagus | Acute esophagitis | Oral anesthetic solutions (eg, viscous lidocaine + benadryl or sucralfate suspension), soft diet, consider empiric antifungal | ||
Prostate | Urinary irritative/obstructive symptoms | Alpha-1 blocker, anti-inflammatory, phenazopyridine (caution with antispasmodics) | ||
Stomach | 50–60 | 65–70 | Ulceration, perforation | Surgical management |
Small intestine | 40–50 | 55–60 | Obstruction, perforation, fistula | Surgical management |
Colon | 45–55 | 55–65 | Obstruction, perforation, fistula | Surgical management |
Rectum | 60 | 80 | Proctitis | Mesalamine, sulfasalazine, sucralfate enema, steroid suppositories |
Radiobiological effects of radiation therapy at the cellular and tissue level
RT is a local modality that generally causes toxicity limited to the area of treatment (with some caveats). The effects of RT on normal tissues generally result from the depletion of a cell population by cell killing. The cells of normal tissue are not independent units but rather form a complete integrated structure. Response to RT is governed by 3 main factors: (1) inherent cellular radiosensitivity, (2) kinetics of the tissue, and (3) organization of cells within the tissue. Visible damage to an organ becomes evident only if a sufficient proportion of cells are killed. As mentioned previously, cell death after irradiation occurs when cells try to divide. In tissues with rapid turnover, damage can become evident quickly—in a matter of hours (as in gastrointestinal [GI] mucosa) or days (skin). This gives rise to the concept of “early” responding tissues. “Late” responding tissues have toxicity that occurs after a delay of months or years and predominantly in slowly proliferating tissues, such as the lung, kidney, heart, liver, and the central nervous system (CNS). Acute damage is generally repaired quickly secondary to rapid proliferation of underlying stem cells. Late effects, in contrast, may improve but never completely repair. A late effect generally results from a combination of vascular damage through radiation endarteritis in combination with a loss of parenchymal cells. Radiation endarteritis leads to significant atherosclerotic disease several years after initial therapy, in the areas that previously received RT. If an intensive radiation regimen is used with subsequent depletion of the stem-cell population below levels needed for tissue repair, an early reaction in a rapidly proliferating tissue may persist as a chronic injury. This is termed a consequential late effect. An example of this would be necrosis or fibrosis of the skin that occur consequent to severe radiation dermatitis and acute ulceration.
Another concept that is important in understanding radiation toxicity is the idea of an underlying organ’s makeup, namely its functional subunits. The tolerance of normal tissues to radiation is dependent on the ability of clonogenic tissue progenitor cells to maintain a sufficient number of differentiated cells that are appropriately structured to maintain organ function. The structural organization of a tissue is crucial in determining the relationship between survival of clonogenic progenitor cells and organ function versus failure. Structurally defined functional subunits can include the hepatic lobule, the renal nephron, or the pulmonary acinus. Structurally undefined functional units are seen in the cutaneous skin where re-epithelialization of a denuded area can occur either from surviving clonogens within the affected area or by migration from adjacent areas. In the case of organs such as the lung, liver, and kidney, a certain portion can be treated without significant dysfunction. The subunits in these tissues work in parallel to carry out the physiologic function. This is in contrast to serial tissues, such as the spinal cord, where disruption of a portion of the tissue affects all downstream activity.
RT is generally given as a number of small doses on a daily basis, rather than one large fraction (although this does occur as well and is generally referred to as radiosurgery). Dividing a dose into a number of smaller doses, or fractions, allows normal tissues to be spared. This is due to repair of cellular damage that occurs each day. Normal cells typically have better repair mechanisms than those of cancer cells, and RT is used to exploit this difference. Prolonging overall treatment time and decreasing fraction size will generally decrease the severity of “early” effects. Increasing the dose of radiation per fraction to normal tissue may not affect the patient acutely but results in an increased risk of “late” effects several months to several years later. A review of classically defined radiation tolerance is shown in Table 1 . The TD 5/5 and TD 50/5 in Table 1 refer to radiation doses that would be expected to cause a 5% or 50% rate of the given complication or toxicity at 5 years.
Organ | TD 5/5 (Dose in Gy) | TD 50/5 (Dose in Gy) | Clinical Endpoint | Management Considerations |
---|---|---|---|---|
Kidneys | 23–50 | 28–40 | Nephritis | Expectant/dialysis |
Bladder | 65–80 | 80–85 | Bladder contracture/volume loss/refractory hematuria | Consider hyperbaric oxygen (HBO) |
Bladder | Acute cystitis/spasm | Analgesics (eg, phenazopyridine) and/or antispasmodics (eg, oxybutynin) | ||
Femoral head | 52 | 65 | Necrosis | —— |
Skin | 55–70 | 70 | Necrosis, ulceration | Wound/burn management (silvadene, antibiotics, skin grafting if severe) |
Skin | Acute desquamation | Moisturizing creams, hydrocortisone cream, hydrogel wound dressing, consider empiric antibiotics | ||
Bone (mandible) | Osteoradionecrosis | Surgical debridement, antibiotics, consider HBO | ||
Oral mucosa | 60 | 75 | Ulceration, fibrosis, mucositis | Wound care, oral hygiene and baking soda mouthwash, gelclair, narcotics, topical anesthetics, consider empiric antifungal |
Brain | 45–60 | 60–75 | Necrosis, infarction | Corticosteroids, mannitol, neurosurgical evaluation |
Spinal cord | 47–50 | 70 | Myelitis, necrosis |
|
Brachial plexus | 60–62 | 75–77 | Plexopathy, nerve damage | HBO questionable, high-dose steroids questionable, physical therapy |
External/middle ear | 30–55 | 40–65 | Acute/chronic serous otitis | Earwax removal (Debrox), pseudoephedrine, myringotomy in severe cases |
Parotid | 32 | 46 | Xerostomia | Pilocarpine, artificial saliva, antibacterial mouthwash, dental gum |
Lung | 18–45 | 25–65 | Pneumonitis | Corticosteroids, antibiotics |
Heart | 40–60 | 50–70 | Pericarditis | Corticosteroids, nonsteroidal anti-inflammatory |
Esophagus | 55–60 | 68–72 | Stricture, perforation | Dilation |
Esophagus | Acute esophagitis | Oral anesthetic solutions (eg, viscous lidocaine + benadryl or sucralfate suspension), soft diet, consider empiric antifungal | ||
Prostate | Urinary irritative/obstructive symptoms | Alpha-1 blocker, anti-inflammatory, phenazopyridine (caution with antispasmodics) | ||
Stomach | 50–60 | 65–70 | Ulceration, perforation | Surgical management |
Small intestine | 40–50 | 55–60 | Obstruction, perforation, fistula | Surgical management |
Colon | 45–55 | 55–65 | Obstruction, perforation, fistula | Surgical management |
Rectum | 60 | 80 | Proctitis | Mesalamine, sulfasalazine, sucralfate enema, steroid suppositories |
Toxicity by organ system
Skin and Soft Tissue Toxicity
Acute radiation toxicity of the skin generally has a time course of several days to weeks after beginning RT. Before the advent of modern megavoltage linear accelerators, acute skin toxicity was the most common dose-limiting factor. Clinical signs of acute radiation toxicity can include erythema, edema, and hyperpigmentation. At higher doses, both moist desquamation and dry desquamation can occur. Management generally consists of cleansers, moisturizers, petrolatum-based products (for dry desquamation), topical steroids (somewhat controversial), wound cleaners/epithelial stimulants, and hydrocolloid dressings. Skin reactions are typically self-limited and will resolve over a matter of a few weeks’ time. In some cases with severe desquamation, topical burn medication such as silvadene cream may be required, and patients may need to be admitted for wound management and antibiotics for a potential superinfection.
Fibrosis is one of the most common chronic skin and soft tissue toxicities that occur in patients who have undergone RT, with a time course that begins several months to a few years after completing RT. This may occur in the absence of a severe acute skin reaction. The etiology is thought to be a characteristic RT-induced endarteritis with vascular and capillary damage. Chronic tissue hypoxia leads to fibroblast proliferation and eventual scarring and fibrosis of the affected tissue. As with any late effect, dose, volume, and RT fractionation can affect the eventual clinical outcome. Other factors such as previous surgical manipulation and underlying medical comorbidities, such as diabetes, hypertension, and vascular disease, can also affect tissue oxygenation and increase the prevalence of fibrosis. Fibrosis is generally permanent, and the severity may increase with further follow-up. Clinical signs can include cutaneous induration, lymphedema, joint restriction, ulcerations, atrophy, telangiectasia, and photosensitivity. Skin and soft tissue necrosis is generally managed with supportive care. A combination of pentoxifylline (PTX) and vitamin E has been examined for treatment of fibrosis. Initial studies in swine models demonstrated an approximate 50% decrease in the dimensions of fibrosis. This was confirmed in a small study of breast cancer patients given 800 mg/d of PTX and 1000 U/d of Vitamin E. A statistically significant regression in radiation-induced fibrosis was noted in patients taking both medications for 6 months. Nevertheless, the authors concluded that these results require confirmation in a larger study. Longitudinal studies suggest that a long duration of therapy (12–24 months) is required for maximal regression of fibrosis. Hyperbaric oxygen (HBO) treatment may also be effective for soft tissue necrosis. In one review of 23 patients with soft tissue or bony necrosis of the chest wall, HBO was an important adjunct to surgical debridement, with or without flap placement, and improvement was noted in the majority of patients.
Pulmonary Toxicity
The pulmonary toxic effects of RT can have devastating and longstanding consequences, and this risk must be considered during treatment planning for lung cancer, breast cancer, and other thoracic malignancies. Pulmonary toxicity often presents with an acute inflammatory phase, which may be self-limited and improve with supportive measures, although severe cases may prove fatal. The later onset of RT-related pulmonary fibrosis (PF), which includes fibroblast proliferation and the destruction of normal lung architecture, has more lasting consequences.
Acute pulmonary toxicity that occurs in a previously radiated lung is referred to as radiation pneumonitis (RP). RP generally occurs within 1 to 3 months following completion of RT. The underlying etiology and pathophysiology of RP are related to a cytokine-mediated signal cascade with proinflammatory and profibrotic factors. Some preclinical studies suggest a role for transforming growth factor beta (TGF-β) and interleukin-6 (IL-6) as well as other cytokines in the development of RP. Important factors in determining the risk of RP include volume of lung irradiated, the dose per RT fraction (to functioning lung), and the use of concurrent or sequential chemotherapy. Although several metrics have been evaluated to predict for RP, the V20, or volume of lung receiving at least 20 Gy, is the most frequently used parameter. Baseline poor pulmonary capacity and smoking may predict an increased risk of RP. Individualized biologic factors are important, and serum TGF-β levels may help identify patients at risk of RP. A study from Duke University was successfully able to escalate the RT dose in patients who had lower TGF-β levels.
The diagnosis of RP is generally made with an appropriate clinical history in a patient who has undergone previous RT to the chest (ie, lung cancer, breast cancer, or lymphoma). The differential diagnosis includes infectious pneumonia, pulmonary embolism, and tumor recurrence. The diagnosis of RP may be particularly challenging in lung cancer patients who are at risk for pulmonary compromise due to severe underlying pulmonary dysfunction. Clinical symptoms are nonspecific and include cough (nonproductive or productive of clear sputum), low-grade fever, dyspnea, fatigue, and pleuritic chest pain. Physical examination may reveal crackles or a pleural rub. Imaging very early on in the course of RP may not show abnormalities. Ground-glass opacities that conform to the RT port classically define RP, although this is not a consistent finding. In some patients, a lymphocytic alveolitis involving both adjacent lobes in the ipsilateral lung as well as the contralateral lung has been described after undergoing RT. Symptoms are similar to RP, but radiographically, more diffuse changes are noted. A typical example of acute RP is shown in Fig. 1 .
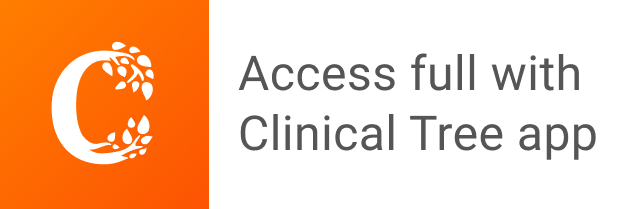