Proton Therapy
The burgeoning interest in proton therapy is related to its potential to improve the therapeutic ratio for many malignancies treated with radiation. This chapter will touch on the rationale, evolving technology, potential applications, efficacy, toxicity, comparative effectiveness, and economic challenges associated with proton therapy.
RATIONALE
Therapeutic Ratio
With any medical intervention there is an optimum balance between the potential to benefit and the potential to harm the patient, called the therapeutic ratio. In radiation oncology, it is the balance between radiation effects on the tumor and radiation effects on normal, nontargeted tissues. This ratio informs every physician and patient decision, but different priorities may mandate different decisions in different clinical situations. For example, in a patient with an advanced paranasal sinus tumor adjacent to the optic nerves and chiasm, one physician might recommend a treatment that minimizes the risk of optic nerve damage at the price of a lower probability of tumor control, while another physician might recommend a treatment with a greater risk of optic nerve damage to provide a greater probability of tumor control. In another example, the potential risks of low-dose radiation exposure to a large volume of the brain tissue may be of greater concern to the parents of a young child than to an elderly patient with a short life expectancy. In evaluating new radiation modalities, the first question is whether there exists a potential to improve the therapeutic ratio.
The Impact of Dose Distribution on the Therapeutic Ratio
A number of factors influence radiation effects, and thus the therapeutic ratio, including total dose, dose intensity (the overall time or number of fractions in which a dose is given), relative biologic effectiveness (RBE), and modifying factors such as chemotherapy, patient age, and comorbidities. But none of these factors is as important as dose distribution—the relative dose to the target compared with the dose to nontargeted normal tissues—because radiation therapy is a nonspecific therapy that affects both normal and cancerous cells. A constant principle in radiation oncology is that the higher or more intense the radiation dose, the greater the probability of tumor control. The risk of treatment-related toxicity is similarly related to the dose and dose intensity to normal tissue, as well as to the volume of the normal tissue exposed to various radiation dose levels. This principle has been demonstrated in many malignancies (i.e., prostate, breast, head and neck) but may not always be apparent when tumoricidal doses cannot be delivered to the target because of excessive risks to normal tissues or when adequate tools for measuring toxicity are not available.
The primary barrier to maximizing local tumor control through dose escalation or intensification is the risk of damaging normal tissues either by delivering too high a dose or exposing too much of the normal tissue to radiation. Whereas tumor control is a dichotomized end point, toxicity occurs as a spectrum of end points over a long period of time in a variety of tissues and can be difficult to measure. When toxicities are easily defined, are functionally significant, and occur early, the risk of the radiation complication is often prioritized over the probability of cure, leading to a low therapeutic ratio because of low disease control rates. Conversely, when toxicities are ill-defined, are difficult to measure, or occur late, disease control will be prioritized. In most clinical settings, there is an opportunity for improvement of the therapeutic ratio by increasing disease control or by reducing toxicity. The most direct means of improving the therapeutic ratio is by reducing the radiation dose to nontargeted tissues, which both reduces toxicity and facilitates dose escalation for increased tumor control: herein lies the rationale for proton therapy.
FIGURE 19.1. The shape of the depth-dose curves for electrons (tan), photons/x-rays (orange), a pristine proton Bragg peak (purple), and a spread-out Bragg peak (blue), composed of multiple pristine Bragg peaks, differs significantly. Compared to photons or electrons, the entrance dose with protons is constant and reduced relative to the target dose and there is no exit dose. The dose fall-off at the end of the proton range is much sharper than for electrons. In summary, with photons, most of the radiation energy is deposited outside of the target, whereas with protons, most of the radiation energy is deposited inside the target.
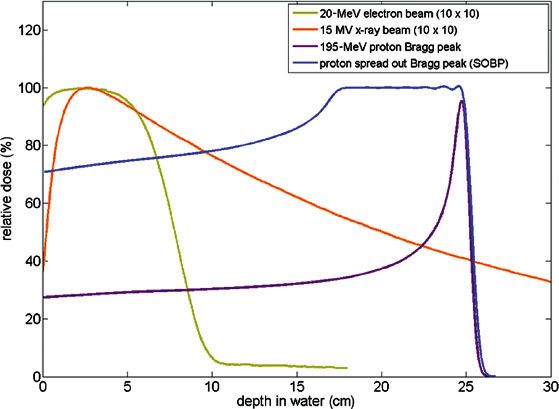
The Problem with X-Rays and the Promise and Challenge of Protons
Most x-rays from an external beam pass through the patient without effect, but some are absorbed as they randomly interact with subatomic particles along their path, initiating a cascade of biochemical reactions that result in cell injury or cell death. X-rays thus leave a track of tissue damage from the skin-surface entrance to the skin-surface exit, much like the track of a bullet. With accumulating x-ray absorptions, the dose deposited along the beam path is attenuated as the number of x-rays available for interactions decreases. This pattern of radiation dose deposition with x-ray-based external-beam radiotherapy (EBRT; Fig. 19.1) is particularly problematic because the dose to tissues along the beam entrance path is always higher than the dose to the target. In addition, along the exit path of the beam, more normal tissues are exposed to doses of radiation only slightly less than the dose to the target. Thus, most of the radiation dose with x-ray-based therapy is deposited in the patient outside of the target.
Many elegant strategies have been developed for offsetting this basic problem (i.e., multiple fields, four-field box, stereotactic radiosurgery and stereotactic radiation therapy [SRT], three-dimensional conformal radiation therapy [3DCRT], intensity-modulated radiation therapy [IMRT], tomotherapy, arc therapy, robotic radiosurgery, etc.), but even with these technologies, radiation to nontargeted tissues is primarily redistributed, rather than reduced, and more radiation dose is deposited outside, versus inside, the target. Because the total dose to a given tissue is one of the key determinants of toxicity, certain toxicities are reduced when using these sophisticated techniques. Nevertheless, it is likely that other toxicities, influenced by the volume of tissue exposed to radiation, will increase by these integral dose redistribution methods as greater volumes of normal tissue are exposed to radiation. Some of the effects related to low-dose exposure to larger volumes of tissue may require more time for clinical manifestation (second malignancies), and some injuries may require more subtle measurement tools for detection (neurocognitive testing), leading to an early underestimation of toxicity with these sophisticated methods.
The pattern of dose deposition with protons differs significantly from that of x-rays. A proton is simply a hydrogen atom that has lost its electron. It has a mass of approximately 1,800 times that of an electron and carries a positive charge. As protons traverse matter, they lose energy primarily through interactions with atomic electrons. Due to the significantly larger mass of protons relative to electrons, protons lose only a small portion of their energy in each interaction (in contrast to x-rays) and experience only small directional changes.
As protons traverse matter, the rate of energy loss in electronic interactions is described by the linear stopping power, S(E), defined as dE/dx, where dE is the mean energy lost by a proton in electronic collisions over a distance of dx in media. A more commonly used function is the mass stopping power, s = S(E)/ρ, which denotes the energy loss dE when protons travel through a distance of dx in a media of density ρ.1
Linear energy transfer, or LET, is a closely related concept to stopping power. Whereas stopping power indicates energy lost by the protons, LET denotes the energy transferred to a medium as the protons traverse it. LET therefore represents the density of energy deposition in media and is directly related to the local RBE of the radiation, similar to its significance in other radiation modalities. At higher energies (e.g., along the entrance path), protons have a small stopping power and low LET values; their LET values increase sharply by up to two orders of magnitude as the proton’s energy decreases just before coming to a complete stop. This phenomenon creates the Bragg peak of radiation dose deposition characteristic of a proton beam, with a small, nearly constant, dose along the entrance path followed by a sharp peak in dose deposition immediately before the protons stop in media. In addition, the LET values rise sharply, corresponding to a sharp increase in RBE values near the end of a proton beam range. Much work has been done to determine in vitro and in vivo proton beam RBE values.2 For clinical applications of protons, it is currently recommended that an RBE multiplicative value of 1.10 should be applied to the physical dose.1 Currently, this RBE factor is applied uniformly to the physical dose distribution, without explicitly accounting for the increased RBE values near the end of a proton beam range. There is a rapid rise in RBE during the last several mm3 of the proton range, so that the actual RBE-corrected dose at the very end of the range may exceed the physical dose by up to 25%, thus producing an RBE value up to 1.3 at the very end of the proton range. RBE effects are much more significant in heavier ions like carbon due to their significant variations along beam paths; nascent carbon ion treatment-planning systems are attempting to account for these variations in RBE along the carbon beam path.4
In addition to energy loss through electronic collisions, high-energy protons also experience nuclear interactions in media. Such interactions, from the perspective of clinical proton therapy, remove protons from the initial proton fluence and produce secondary protons and heavier particles, such as deuterons, tritons, 3He, and α; these particles contribute only a small percentage of the dose along the beam path.5 Although negligible, most of the dose from the neutrons produced in these nuclear interactions is deposited downstream from the stopping point of the proton beam, beyond the Bragg peak. The neutrons produced by protons within the patient, as well as those produced in the beam delivery mechanical components, have been under intense investigation for their potential secondary cancer-inducing effects. Hall6 raised concern that such scattered neutrons may increase the incidence of secondary tumors in patients treated with historical proton therapy systems, similar to the transition from conventional 3DCRT to IMRT.7 Much research has been performed estimating secondary cancer risks from contemporary proton therapy systems using either scattering-beam techniques or scanning-beam techniques. A review of literature on this subject finds consistent advantages of proton therapy over photon therapy techniques in the form of reduced secondary cancer risks in the treatment of medulloblastoma, prostate cancer, and liver cancer.8
Accordingly, the rationale and promise of protons, compared with x-rays, lie in the significant reduction in dose to nontargeted tissues along the entrance path and the absence of exit dose. This reduction in dose (physical and RBE dose) to normal tissues should translate into a lower risk of complications for a given target dose and probability of tumor control (i.e., an improved therapeutic ratio compared with x-rays). A lower risk of complications could be leveraged for dose escalation or intensification to yield higher disease control in certain settings or for hypofractionation to reduce costs or increase tumor control in other settings.
TECHNOLOGY
Beam Production and Transport
Protons are produced either from hydrogen gas obtained from electrolysis of deionized water or from commercially available high-purity hydrogen gas. Application of a high-voltage electric current to the hydrogen gas strips the electrons off the hydrogen atoms, leaving positively charged protons. The protons are then accelerated to energy applicable for clinical proton therapy with either a cyclotron or a synchrotron (Fig. 19.2). Energies on the order of 250 MeV are required for penetrating approximately 32 cm into tissue. Cyclotrons produce a continuous beam of nearly monoenergetic and unidirectional protons. The beam must then be degraded to meet specific requirements for each treatment field in each patient. This process takes place shortly after the beam exits the cyclotron in a device composed of a material of variable thickness with a low atomic number known as the “energy degrader.” The proton beam exiting the energy degrader will have an energy spread centered around the desired final beam energy and direction variations that reduce the quality of the final treatment beam. An energy selection system (ESS), consisting of energy slits, bending magnets, and focusing magnets, is then used to eliminate protons with excessive energy or deviations in angular direction. Cyclotrons can produce a large proton beam current of up to 300 nA and thus deliver proton therapy at a high dose rate using the traditional double-scattering technique.
Synchrotrons, however, produce proton beams of selectable energy, thereby eliminating the need for the energy degrader and energy selection devices. A proton pulse exiting a preaccelerator, with energy typically up to 7 MeV, is injected into the ring-shaped accelerator. Each complete circuit of the proton pulse through the accelerator ring structure incrementally increases the proton pulse energy. When the desired beam energy is reached, the proton pulse is extracted from the accelerator. The time segment between pulses depends on the final energy required of the proton beam, ranging from subseconds to several seconds for the highest beam energy. The pulsed nature of the beam introduces additional complexity in certain treatment delivery scenarios, such as gated treatment of mobile targets and intensity-modulated proton therapy (IMPT).9 Beam currents from synchrotrons are typically much lower than with cyclotrons, thus limiting the maximum dose rates that can be used for patient treatment, especially for larger field sizes. The maximum dose rate available from a commercially available synchrotron-based proton therapy system for a 25 × 25-cm2 field has been specified at 0.8 Gy per minute.10 The elimination of the energy degrader and selection system removes a major source of radiation production in the accelerator vault. A synchrotron vault is therefore accessible immediately after the beam is stopped for maintenance, whereas for a cyclotron vault, approximately 30 minutes is necessary to allow the activated parts of the accelerator and ESS to “cool down” before maintenance can be performed, or longer if access to internal parts of the cyclotron is required. The shielding requirements for cyclotrons are higher than for synchrotrons as well due to the high radiation produced by the ESS. However, the overall footprint of a cyclotron vault, including the additional shielding required, is actually similar to or smaller than that of a synchrotron vault because of the smaller physical dimensions of cyclotrons.
The proton beam, whether exiting the ESS for a cyclotron-based system or exiting the accelerator for a synchrotron-based system, is transported to the treatment room(s) via the beam transport system. Maintenance of beam focusing, centering, spot size, and divergence throughout the beam transport system is critical to maintaining a high-quality proton beam for treatment delivery. Paganetti et al.11 performed Monte Carlo calculations of the dosimetric effect of proton beam energy spread, spot size, and angular energy spread on the shape of the Bragg peak. They found that small deviations in these parameters from their nominal values will result in a widening of the Bragg peak and increased entrance doses. Beam transport systems in clinical proton facilities therefore include bending and focusing magnets and beam profile monitors so that the proton beam quality may be monitored and adjusted (“tuned”) as it is transported through the beam transport system.
Recent progress in accelerating and control technologies has led to new designs of proton therapy systems in the form of single-treatment-room systems with small cyclotrons that may be mounted on a gantry, as well as dielectric wall accelerators (DWAs) and laser-accelerated accelerators.12 Single-room proton therapy systems are currently available from a number of commercial vendors, each with its own strengths and weaknesses, although none was in clinical operation at the time this chapter was written. DWA and laser-accelerated systems are currently under development. One of the primary drivers for development of these single-room proton therapy solutions is facility cost reduction.
FIGURE 19.2. Proton therapy system with cyclotron, energy selection system, beam line, gantry, and nozzle. Scattering is illustrated in the nozzle on the left and scanning in the nozzle on the right.
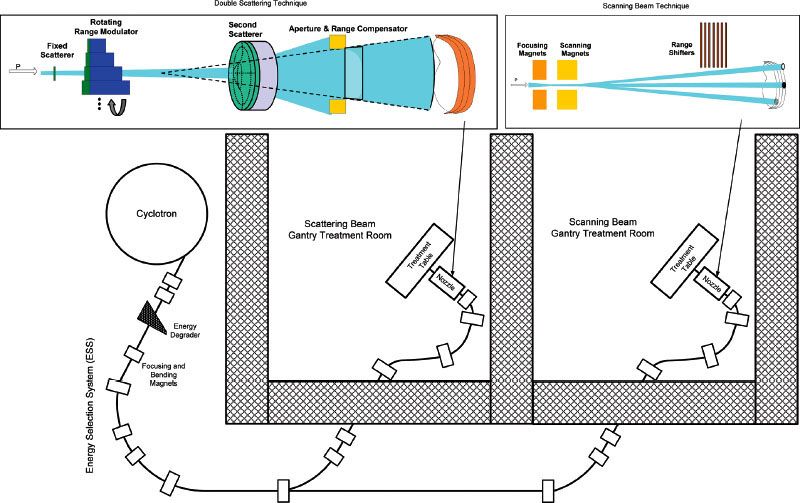
Beam Delivery
The proton beam exiting the transport system (Fig. 19.2) is a pencil-shaped beam with minimal energy and direction spread. The beam has a small spot size in its lateral direction and a narrow Bragg peak dose in its depth direction. Two basic techniques have been developed to convert this narrow pencil beam into a dose distribution suitable for treatment of a 3D target, broadly categorized into the “scattering technique” and the “scanning technique.” These techniques are implemented in the proton treatment “nozzle,” or treatment head.
The scattering technique aims to produce a dose distribution with a flat lateral profile, similar to what is achieved in linear accelerator–produced 3D conformal x-ray beams, but a depth dose with a low entrance dose and a plateau region, followed by the sharp fall-off of doses to zero. The depth-dose curve, with a plateau of adequate width to cover the full thickness of the target, is produced by summing a number of Bragg peaks, each with progressively reduced maximum energy and range in the patient. The weights of the individual Bragg peaks are optimized to achieve a flat top region of the depth-dose curve. A constant energy proton beam may be used for such treatments, with range-reducing materials inserted into the beam path to create subsequent Bragg peaks of reduced ranges. Range modulation wheels consisting of variable thicknesses of “acrylic glass” (polymerized methyl methacrylate) or graphite steps are traditionally used for this purpose.11 The proton beam travels through the variable-thickness steps, with each step creating a Bragg peak of a precalibrated range. The widths and thicknesses of the modulation wheels are calibrated to achieve a flat depth dose, or “spread-out Bragg peak” (SOBP). The width of the SOBP is controlled by turning the beam off when a prescribed width is reached. Alternatively, a “ridge filter” may be used to create an SOBP,13 with the advantage of eliminating sensitivity to organ motion in the formation of the SOBP but the added complexity that each ridge filter is designed to achieve a single SOBP width.
The scattering technique produces a large, flat lateral dose profile through physical scattering filters in the proton beam path.11 Either a single scattering filter may be used, creating a wide beam with a Gaussian dose profile (single scattering beam), or a second scattering filter can be added into the beam that flattens the beam to create a flat lateral dose profile (double scattering beam). Apertures fabricated out of brass or other metal are used to confine the treatment field to the target. A tissue-equivalent range compensator (or bolus) is designed to adjust the beam range throughout the field to conform to the distal profile of the target.14
In the scanning technique, as the pencil beam exits the transport system, it is magnetically steered in the lateral directions to deliver dose to a large treatment field.10,15–17 The proton beam intensity may be modulated as the beam is moved across the field, resulting in the modulated scanning beam technique, or IMPT, delivery. Current implementation of IMPT uses the so-called spot scanning technique, in which the beam spot is moved to a location within the target and the prescribed dose delivered to the spot, before it is moved to the next spot to deliver its prescribed dose. In the beam axis direction, IMPT treatments are delivered using the layer-stacking technique. A pencil beam with a pristine peak is scanned through the deepest layer of the target to deliver the intensity-modulated dose distribution for the layer before a range shifter—effectively an energy degrader of predetermined thickness—is inserted into the beam path to deliver dose to a depth immediately proximal to the deepest layer. Doses to each subsequent layer are delivered by inserting additional range shifters. The size of the spot, represented by the sigma of the Gaussian function describing the pencil-beam profile, is a critical parameter of the pencil beam. Smaller beam sigma values allow intricate sculpting of the intensity-modulated dose distribution, at the cost of increased control system complexity and delivery time; larger beam sigma values result in greater lateral penumbra of the delivered dose distribution and reduce the dose gradient achievable at interface regions of target and critical organs.
Similar to the interplay effect reported for IMRT,18–21 scanning techniques are sensitive to organ motion because moving the pencil beam across the treatment field for dose delivery to a given layer and inserting range shifters for dose delivery to subsequent layers are time consuming. Additional motion mitigation measures, such as breath holding, gated therapy, and abdominal compression, may be necessary to minimize the dosimetric effects of organ motion.
Treatment Planning
Treatment planning for proton therapy requires a volumetric patient computed tomography (CT) scan dataset. The CT Hounsfield Unit numbers are converted to proton stopping-power values for calculating the proton range required for the treatment field.22,23 Unlike the relatively reliable conversion of CT numbers to relative electron density for photon dose calculations, errors and uncertainties in the conversion of CT numbers to proton stopping power in proton dose calculations translate linearly into proton range calculation uncertainties and errors. In clinical practice, these uncertainties are handled during the treatment planning process by bracketing the intended SOBP with a distal margin beyond the target and a proximal margin before the target in the range calculation of each treatment field.24 Other considerations in determining the values for distal and proximal margins include target motion, daily setup errors, beam delivery uncertainties, and uncertainties in patient anatomy and physiology changes throughout treatment that could affect the water-equivalent depth of the target. In the lateral direction of the beam’s eye view (BEV) of a proton field, planning target volume (PTV) margins are necessary to accommodate setup errors and target movements, identical to their usage in x-ray treatments. It is therefore worth noting that the concept of PTV, as defined in the various International Commission on Radiation Units and Measurements (ICRU) reports,1,25,26 does not strictly apply to proton therapy. Generally, a PTV expansion, with either uniform or nonuniform margins, is used for x-ray planning, which accommodates maximum target motion and setup error in each of the patient axes (longitudinal, lateral, and anterior/posterior). In contrast to x-ray planning, the PTV for proton therapy is specific for each treatment field. Lateral margins are identical to traditional definitions, but the distal and proximal margins along the beam axis are calculated to account for proton-specific uncertainties. The lower entrance dose and absence of exit dose of a proton beam afford proton therapy practitioners the additional flexibility to select beam angles where lateral target motion and setup error are minimal; thus, the PTV expansion margin may be less for a given proton beam than would be required in IMRT to accommodate for uncertainties for all beam angles. State-of-the-art proton therapy dose calculations use pencil-beam algorithms,27–30 which model proton interaction and scattering in various heterogeneous media of the beam path, including the nozzle, range compensators, and the patient. Monte Carlo calculations have been used to study the accuracy of such dose calculation algorithms, with results indicating errors near tissue interfaces, particularly near interfaces of media differing significantly in density and composition, such as air cavity and bone in head and neck treatments.31–33 A number of fast Monte Carlo calculation algorithms have been proposed to improve proton therapy dose calculation accuracy,34–36 although none is currently available from commercial treatment-planning system vendors.
POTENTIAL PROTON THERAPY APPLICATIONS
Many publications have reported significant differences in dose distribution with proton treatment plans compared with x-ray-based treatment plans in a wide range of malignancies and benign lesions throughout the body, including the eye,37 brain,38,39 sinonasal structures,40,41 oropharynx, nasopharynx, skull base, lung,42,43 lymphoma,44,45 pancreas,46 esophagus,47 bladder, rectum, pediatric malignancies, prostate,48,49 cervix,50 breast,51,52 sarcomas, and standard target volumes such as pelvic lymph nodes53 and craniospinal irradiation.54 In all cases there is a striking advantage with protons for reduction in the volume of nontargeted normal tissue receiving low- to medium-range radiation doses. In some cases, there is also a reduction in the volume of nontargeted tissue receiving moderate- to high-dose irradiation. With double-scattered proton delivery modes currently in common usage, the target dose homogeneity and conformality index can sometimes, but not always, be inferior to that of IMRT. With scanned proton delivery modes, IMPT plans generally have not only significant reductions in low and moderate integral doses but also improved dose homogeneity and conformality indexes when compared with IMRT. Each case within each tumor type is different, and until comparative plans are performed, it may not be clear whether protons will be helpful or whether IMPT would be useful. At this stage in the development of proton therapy, there are no clear class solutions to treatment planning. In addition, the full potential for dose distribution improvements with protons has not been realized because of uncertainties in both treatment-planning algorithms and delivery modes. Strategies for motion management and quality assurance necessary to implement advanced proton delivery modes into routine practice are not fully developed. Finally, the clinical impact of some patterns of dose distribution improvements achievable with proton therapy may not be anticipated at this time and/or may require time, careful trial design, and special assessments to define.
Currently, proton therapy is a rare medical resource best used in situations where outcomes with commonly available radiation strategies present opportunities for improvement in the therapeutic ratio via improvements in dose distributions. Listed in Figures 19.3 through 19.10 are eight clinical settings that exemplify particular kinds of opportunities for proton therapy. The proton plans are all double-scattered mode, as few practices today are able to deliver IMPT. The first two examples (Figs. 19.3 and 19.4) represent opportunities where reductions in relatively high doses to critical structures make it possible to deliver a sufficiently high target dose with proton therapy to make disease control possible in cases not often cured with photon therapy. The next three examples (Figs. 19.5 through 19.7) are opportunities to reduce early functional and potentially fatal late toxicities with proton therapy by reducing the volume of tissue exposed to low and moderate radiation doses. Figures 19.8 and 19.9 represent opportunities to increase the effectiveness of combined modality therapy by increasing dose or dose intensity of either chemotherapy and/or radiation therapy made feasible through reducing moderate-dose irradiation to critical structures subject to acute toxicity. The last example (Fig. 19.10) represents an opportunity to improve the therapeutic ratio in a tumor generally considered to be well treated with sophisticated x-ray techniques; in this case, a small reduction in toxicity can be leveraged to facilitate hypofractionation, which may both enhance disease control and reduce health care costs without increasing toxicity.
FIGURE 19.3. Axial and sagittal planes from intensity-modulated radiation therapy (IMRT) plans are shown on the left and proton plans on the right for a skull-base sarcoma. In this particular case, the major advantage to the proton plan over the IMRT plan is the sharp gradient between the target and brainstem achievable with the proton plan. The maximum and mean relative doses to the brainstem are 71% and 42% with IMRT compared to 59% and 11% with protons, respectively. The lower maximum and mean relative doses to the brainstem permit delivery of a higher dose to the clivus sarcoma target. In addition, there is a substantial reduction in low-dose exposure to nontargeted tissue such as the posterior fossa, spinal cord, and nasal cavity, which might result in more acute tolerance of treatment or fewer late neurocognitive sequelae.
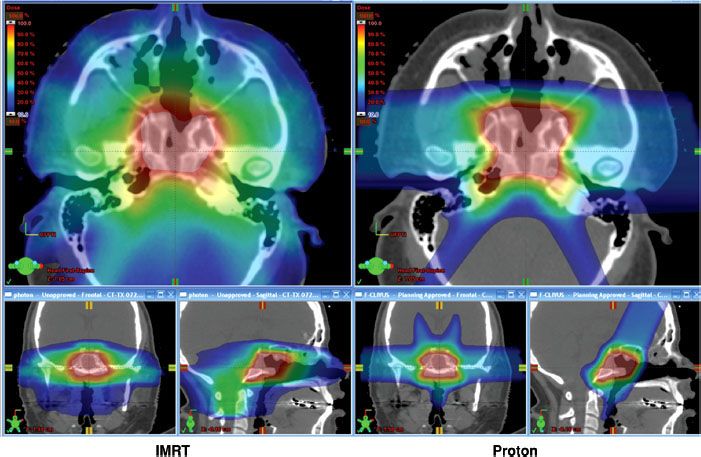
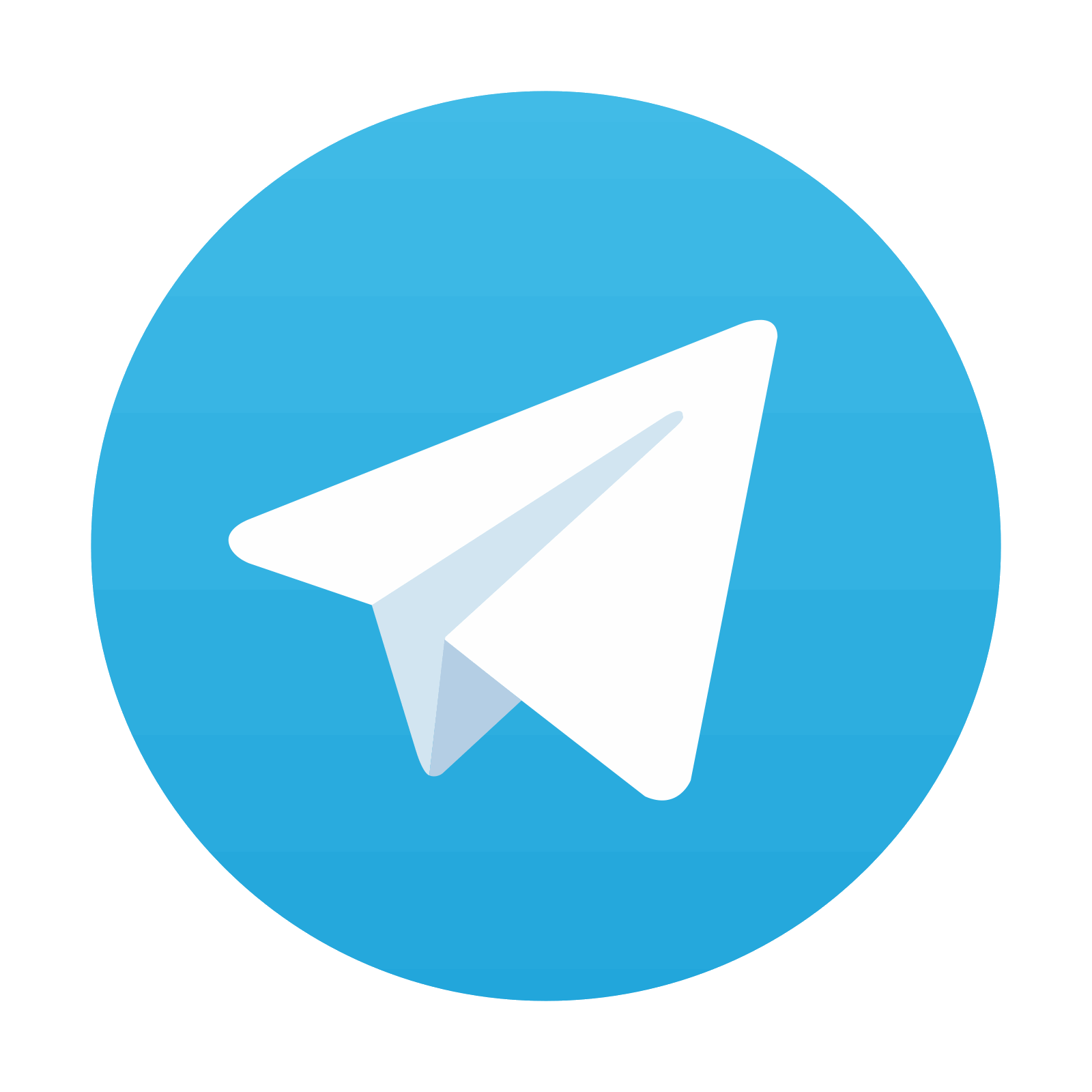