Photodynamic Therapy
The first report of cytotoxicity (in paramecium) observed by combining a drug (acridine) and visible light can be traced to the medical student Oscar Raab working in the laboratory of Herman von Tappeiner in 1900.1 This observation laid the foundation for the 1903 descriptions by von Tappeiner and Jesioneck who combined topical eosin and visible light for the treatment of a skin tumor.2 Modern investigations of photodynamic therapy (PDT) have since been attributed to Lipson et al.3 in 1960, with the discovery of hematoporphyrin derivative (HPD) by Samuel Schwartz,4 a water-soluble mixture of porphyrins and a series of preclinical and clinical investigations led by Dougherty et al.5
Despite its deep historical origins and modern research investigations dating back 40 years, the clinical application of PDT remains limited to specific clinical situations. In part, this has been due to the superficial depth of cytotoxicity achievable with past photosensitizers and light delivery techniques. It is also due to the complexity of its application, requiring familiarity with safe photosensitizer administration coupled with the technical requirements for effective light delivery and the optical expertise to prescribe and deliver an activating light energy in a selective manner.
Despite these disadvantages, there are several compelling reasons to evaluate PDT as a major therapeutic approach in the management of cancer. Central to this is its unique mechanism of action allowing for nonoverlapping toxicities with traditional cancer therapeutics. As such, PDT does not exclude the subsequent administration of these treatment modalities. Technical advances with interstitial light delivery techniques (and advancements in modeling its dosimetry6) along with the clinical development of photosensitizers capable of absorbing and being activated at longer wavelengths now offer the potential for more penetrating cytotoxicity.7 Unlike traditional chemotherapeutics and ionizing radiation therapy, there has also been a paucity of any long-term genotoxic effects with the use of PDT, an observation consistent with several in vitro studies.8–10 The explosion in our understanding of tumor biology and the influence of the microenvironment has also provided tremendous insights into the development of novel strategies to optimize the clinical efficacy of PDT, including its combination with biologic therapeutics. This also includes the potential for PDT to enhance the effects of traditional cancer therapeutics and its promising role to more effectively induce adaptive cell-mediated immunity.
TABLE 27.1 FACTORS AFFECTING THE EFFICACY OF PHOTODYNAMIC THERAPY
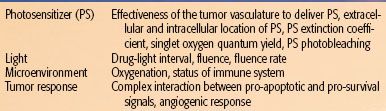
PRINCIPLES OF PHOTODYNAMIC THERAPY
Photosensitizers
PDT represents a treatment modality that combines the selective photochemical activation of photosensitizers with electromagnetic radiation in the visible energy range (i.e., light). Photosensitizers (PS) may be introduced into the cancer patient either systemically, topically, or injected locally, but it is its chemical structure that can significantly influence the effectiveness of a PDT treatment. These include influencing its biodistribution and subcellular localization along with how efficiently it absorbs light (referred to as its molar extinction coefficient) to generate reactive oxidative species (ROS), including singlet oxygen (referred to as its quantum yield) (Table 27.1). A PS with a low molar extinction coefficient will require large concentrations of the PS and light energy to be effectively delivered for photoactivation.
There are now a multitude of PS that have been generated with many under preclinical evaluation and several receiving regulatory approval for clinical application in the United States, European Union, and other countries. The first PS to receive regulatory approval was a semipurified preparation of HPD known as Photofrin (porfimer sodium). Porfimer sodium represents a complex mixture of hematoporphyrin oligomers whose chemical composition has been difficult to fully characterize and reproduce consistently. It has an absorption peak at 630 nm with a relatively low molar extinction coefficient, thus requiring large concentrations of drug and light energy (fluence) to be delivered. It has also been shown to have a prolonged risk of skin photosensitivity reflecting its relative lack of tumor selectivity; and while used routinely in clinical practice, these disadvantages have spurred ongoing PS development.
Several ideal characteristics have been well articulated in the area of PS development. These include a PS that has a well-established structure, ideally a pure compound with a constant composition and a stable shelf-life. Without light activation, it should have little toxicity and tumor specificity when administered. With light, a PS with spectral absorption peaks that demonstrate a high extinction coefficient will be more efficiently activated. However, it is not entirely clear if this is always desired. Strong PS absorption at a specific wavelength can further contribute to reduced light penetration, a phenomenon referred to as self-shielding. Moreover, potent PS such as temoporfin/m-THPC (Foscan) that thus require little drug and light for its efficient activation have been associated with significant complications necessitating even more vigilance to the light dosimetry (see below).11 The wavelengths of a photosensitizer’s absorption peaks also influences how the PS will be used clinically, with absorption at longer wavelengths (i.e., 700 nm range) offering deeper light penetration in human tissues. Lastly, some PS may undergo a process of photobleaching, whereby the PS in turn reacts with the singlet oxygen or other ROS created in the photoactivation process altering its ability to act as a PS. Typically, photobleaching decreases a photosensitizer’s reactivity, which may or may not be desirable depending on the context of its clinical application.
Structurally, photosensitizers are generally classified as porphyrin-based or nonporphyrins with the former sharing a common backbone that consists of the tetrapyrrole ring. Other structural backbones that have demonstrated photosensitizing capabilities include the presence of four phenol rings and polycyclic ring compounds based on the pyrrole ring especially the tetrapyrrollic PS. Extensive reviews are available regarding the specific physicochemical properties (i.e., primary structures, the presence of complexed heavy metals, and specific side chain substitutions) and the impact on its systemic biodistribution, cellular uptake, and photosensitizing properties.7 In general, the ability for hydrophobic compounds with two or less negative charges can still cross the plasma membrane. Otherwise, intracellular uptake is through active endocytosis. The charge of the hydrophobic PS can also influence where a PS localizes with cationic charges (positive), tending to localize to the mitochondria and anionic PS with a net charge of negative two or greater tending to localize in the lysosomes.12 Cationic PS localizing to the mitochondria have been suggested to be more effective in mediating direct cytotoxicity.13
Although tumor specificity is in part mediated by selective light administration and its natural energy attenuation, specific extracellular and intracellular PS delivery is felt to further contribute to this process. Human tissue studies in patients receiving porfimer sodium-mediated intraperitoneal PDT have confirmed PS selectivity, even if narrow.14 Systemically administered photosensitizers, especially those with the tetrapyrrole backbone, will associate with serum proteins, including albumin and low-density lipoproteins (LDL). The association with LDL proteins has been suggested to be a potential mechanism that may contribute to specific tumor localization.15 In this model, it has been proposed that tumor selectivity occurs due to a preferential up-regulation of LDL receptors in tumor cells due to the rapid plasma membrane turnover rate and the need for constituents in its biosynthesis. Alternatively, the functionally altered tumor vasculature resulting from overexpression of vascular endothelial growth factor16 and its impaired lymphatic clearance of the extravasated PS have also been advanced as a working model.17 Although this may lead to increased PS retention, the extracellular distribution of the PS can be inhomogeneous and can impact the effectiveness of a PDT treatment. Korbelik and Grosl18 demonstrated that direct tumor cell killing was a function of the distance from a tumor’s vascular supply. In turn, photosensitizers that tend to be located in the intravascular space can increase the effectiveness of PDT through mediating vascular damage and tumor infarction.19
The subcellular localization of a PS can similarly influence the mechanism of cellular injury and has been well studied and recently summarized.20 In general, photosensitizers that localize to the plasma membrane and lysosomes are likely to cause injury by necrosis. Those localizing to the mitochondria and endoplasmic reticulum are likely to initiate cell death by way of apoptosis.5,20,21 However, mitochondrial injury can also mediate a necrotic cell death with severe inner mitochondrial membrane damage.20
Most photosensitizers tend not to accumulate in the nucleus, possibly explaining the paucity of genotoxicity and observed carcinogenesis.8,22 Although DNA damage has been reported in cell culture experiments for various photosensitizers,8–10,23 especially for 5-aminolevulinic acid,23,24 efficient DNA repair has also been observed, suggesting that the damage may not be sufficient to overwhelm a cell’s repair capacity.10,25 As PDT can mediate cell death through non-DNA targets, the potential mutagenic effects of any DNA injury is likely to be further limited by its cell death.
FIGURE 27.1. Jablonski energy diagram following type II photosensitizer activation.
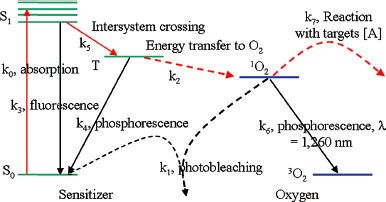
Photosensitizer Activation
Following photosensitizer administration, the drug–light interval (DLI) that is prescribed warrants consideration. Preclinical studies have demonstrated that varying the DLI can influence both the PS extracellular and intracellular localization.19,26 In general, longer DLI will promote PS extravasation and intracellular uptake, provided it has a sufficient long pharmacokinetic lifetime. However, this passive targeting of either the vascular (short DLI) or tumor compartment can be compounded by the lipophilicity of the PS and which proteins it associates with within the vasculature. It would also appear that targeting both the vascular and tumor compartment with repeat PS administration, combining both a short DLI and a long DLI, can further improve the effectively of PDT. However, the order of the repeat PS administration and activation may be particularly important. Prescribing an initial short DLI that targets the vasculature can create subsequent hypoxia that limits the efficacy of the repeat PS with a long DLI.19
PS activation involves the absorption of wavelength specific energy, causing specific changes in the electron energy states of the PS. Energy absorption can cause a PS electron to move from its ground state to a higher energy level or what is referred to as an excited energy state. While in the excited energy state, transition back to the ground state may occur, with energy released in the form of fluorescence or heat dissipation. Thus, some of the light absorbed by a PS may be re-emitted at a different wavelength, allowing for fluorescence detection of the PS or photodynamic diagnosis (PDD). Figure 27.1 demonstrates the Jablonski energy diagram that depicts the energy transitions that may occur with PDT. Two possible excited energy states may be possible: a singlet state (S1) or the triplet state (T1), which has a longer half-life. The distinction depends on the spin direction of the excited electron relative to its paired electron in the ground state. The triplet state has both electrons parallel to each other, and its ability to return to a ground state emitting fluorescence is impeded due to this spin direction.
Transition to the triplet state through a process referred to as intersystem crossing is critical to the generation of cytotoxic ROS. This is largely due to the longer half-life of the triplet state that increases the probability of interacting with a nearby organic molecule (i.e., in the plasma membrane), generating reactive anions or cations that in turn may react with molecular oxygen generating ROS (referred to as a type I reaction). Alternatively, a PS in its triplet state may directly transfer its energy to molecular oxygen to form an excited state singlet oxygen (1O2) (type II reaction). Both types of reactions may occur simultaneously, though direct singlet oxygen production is felt to be the dominant mechanism of cytotoxicity.27,28
Singlet oxygen within the cell has a limited half-life (estimated to be <0.04 μs) and thus a limited range of diffusion and activity (<0.02 μm).22,29 As such, PS cytotoxicity is limited to its extracellular and intracellular (commonly referred to as subcellular) localization and adjacent potential targets for reactivity.22 For example, photosensitizers that tend to biodistribute or favor being localized in the vasculature will favor an antivascular necrotic mechanism of injury. The specific subcellular localization offers an added degree of specificity to its cytotoxicity, as already described.
Physics of Light Dosimetry
Photodynamic therapy is inherently a dynamic process. All three principal components—photosensitizer, light, and oxygen—interact dynamically over the time period of a PDT prescription.5 As light interacts with human tissue, its energy distribution is influenced by surface reflection and with absorption and scatter at depth. Light scatter in human tissues can be affected by many factors. Tissue architecture, its geometry, and its heterogeneity at the histologic and cellular levels can contribute to photon scattering, limiting the energy deposited at depth. Thus, it is important to recognize that light scatter is likely to be different from one tissue type to another. Although this factor remains to be fully characterized such that it can be accounted for in the prescription of PDT, selecting longer wavelengths for photoactivation can help to reduce photon scatter, thus increasing light penetration.
The deposition of light, or its dosimetry, is determined by the light source characteristics and the tissue optical properties, both on the surface and at depth, in contrast to ionizing radiation. In turn, the tissue optical properties are influenced by the spatiotemporal distribution and concentration of both the photosensitizer and oxygen in the illuminated tissues. During illumination, the light dosimetry also dynamically changes as the photodynamic process consumes oxygen and can also alter the blood flow.30 For some photosensitizers, self-shielding can influence the light dosimetry at depth. Finally, the distribution of a photosensitizer may change as a result of photobleaching, a process whereby the photodynamic modification of the photosensitizer itself typically reduces its ability to further be photoactivated.
Quantifying the distribution of the light that is used in photoactivation is important oncologically and for quality assurance reasons, as in the practice of ionizing radiation. The ability to relate the treatment outcome to the effective light fluence and its rate of delivery (fluence rate) facilitates not only an understanding of its relationship to the treatment outcome but also to any potential toxicities, as areas of high fluence and fluence rates have been associated with treatment toxicities. Several approaches to quantifying light dosimetry may be outlined.
Explicit dosimetry refers to the prediction of a singlet oxygen dose on the basis of measurable quantities that contribute to the photodynamic effect.31 In clinical practice, the quantity most amenable to measurement is the PDT dose, defined as the light energy deposited to a photosensitizer. This quantity is proportional to the product of the absorption coefficient of the photosensitizer and light fluence. The absorption coefficient of the photosensitizer is, in turn, proportional to the photosensitizer concentration. PDT dose calculated in this way is a good predictor of outcome if one is operating in a drug- or light-limited situation when there is ample oxygen supply. To generally account for the oxygen effect, the concentration of reacted singlet oxygen (i.e., the concentration of reactions between singlet oxygen and molecular targets within the tumor cells) needs to be modeled as a function of PDT dose and tissue oxygenation. It has been shown that the reacted singlet oxygen concentration can be expressed as the integration of the product of the PDT dose rate and the photosensitizer’s singlet oxygen quantum yield.32
Implicit dosimetry refers to the use of photobleaching of the sensitizer as a measure of the light dose. For sensitizers where the photobleaching is mediated by singlet oxygen, it can be shown that on the microscopic scale, the fractional photobleaching is indicative of the concentration of singlet oxygen reactions induced by PDT.33 Although the relationship to tissue response is more complex, photobleaching has been shown to be predictive of response in animal models34 and used to design protocols for pain reduction during the treatment of patients.35
Accurate light dosimetry presents significant challenges in the clinic. Although it is relatively straightforward to measure the irradiance of light delivered to the surface of a tissue, the light absorbed by the sensitizer also includes the light scattered by tissue not too dissimilar from ionizing radiation. Unlike ionizing radiation, this scattered light contribution may also occur on a surface and is an important concept to consider in the practice of PDT. In hollow organs or any concave surface with surface secretions that further increase the reflective index, light can be reflected from one surface onto another surface, increasing the effective surface light fluence rate. This effect is often referred to as the integrating sphere effect. In the extreme case, multiple reflections can significantly magnify the fluence rate within a hollow organ. This integrating sphere effect has been extensively modeled in the case of bladder treatment.36 In complex concave mucosal surfaces such as in the head and neck, scattered light photons have been demonstrated to increase the effective fluence rate by factors of three- to fourfold.37,38
Light scattering can also occur within tissues, leading to a significant difference between the incident fluence rate that is delivered and the fluence rate within the tissue. In practice, the integrating sphere effect and multiple scattering within the tissue occur simultaneously. Accurate dosimetry requires accounting for both of these effects, ideally through real-time measurements using an isotropic light photon detector capable of capturing both directly incident and scattered light.39 A comparison of measurements using fiber-based isotropic detectors and photodiode detectors that capture only incident light indicated significant difference in the measured light dose with significant variation in the contribution from scattered light.40 Through measurements with a series of light sources and detectors, tissue optical properties can be assessed. However, PDT treatment can significantly change the optical properties. This requires the ability to assess the optical properties in real time for there to be effective feedback to compensate for the influence of varying light transmission on the deposited light dose within the target tissue.41,42 Recently, the first clinical experiences using an automated 18-channel system accommodating optical fibers for light delivery and monitoring was described in patients treated with temoporfin interstitial PDT.6
Biology of Photodynamic Therapy
Mechanisms of Tumor Cytotoxicity
Photodynamic therapy can induce cytotoxicity through all three death morphologies: apoptosis, autophagy, and necrosis. The multitude of signaling pathways (apoptotic and nonapoptotic) and the molecular interplay between various cell death pathways (balanced with the induction of pro-survival signals) that are activated with exposure of the cell to photodynamic oxidative stress have been the focus of intense investigations and the subject of a recent extensive review.43 Of these modes of cell death, apoptosis is a major pathway of PDT-mediated cytotoxicity and reflects the near ubiquitous ability of PDT to induce mitochondrial injury.44,45
Photodynamic therapy can also induce tumor cell injury through direct damage of the endothelial cells of the tumor vasculature. In turn, thrombus formation and the release of various vasoactive molecules, with an increase in the vascular permeability and deterioration of vascular status, ensue. Leukocyte infiltration further compounds this response, all contributing to secondary hypoxia46 with ischemic tumor death and tumor control.47 Preclinical studies also suggest that beyond direct tumor and vascular cytotoxicity, long-term tumor control can only occur in immunocompetent animals.48
Influence of the Tumor Microenvironment on the Photodynamic Therapy Response
As PDT is dependent on the presence and distribution of a photosensitizer, light, and oxygen, the normal tissue microenvironment within which a tumor is located can significantly determine the treatment response of the tumor to PDT. All three key PDT components are subject to effects from the tissue microenvironment. For example, tumor vascular density, permeability, or perfusion can affect photosensitizer delivery,49 which could undoubtedly contribute to the heterogeneities detected in photosensitizer levels among tumors within and among patients.50–52,53–54 Tumor-associated hypoxia can limit PDT-created damage.55,56–58 Even the distribution of the treatment light is affected by the microenvironment due to differences in the penetration of red light as a function of the oxygenation status of hemoglobin. Compared to deoxyhemoglobin, oxygenated hemoglobin is less absorptive of red light (630 or 650 nm), thereby allowing deeper light penetration in tissues with a higher proportion of oxygenated hemoglobin.59
Much research has been performed on the dependence, as well as the effects of, PDT on tumor oxygenation. With conventional photosensitizers, the photochemical process can consume tissue oxygen faster than its delivery, leading to a hypoxic state that limits PDT-mediated cytotoxicity.60,61–62 Even intratumor heterogeneities in tumor oxygenation can have consequences to PDT-mediated cytotoxicity. This has been shown in murine studies that identify the presence of more severe PDT-created hypoxia in the base of subcutaneous tumors accompanied by a relative protection of this area from clonogenic cell death.58
PDT-mediated vascular effects are another cause of hypoxia during PDT. These effects can take the form of PDT-triggered vasoconstriction.63 Additionally or alternatively, PDT can cause endothelial cell rounding, leading to intracellular gaps64 and activation of the coagulation cascade. Ischemia then results, as leukocyte and platelet aggregation with secondary thrombosis within the vessels can further alter the blood flow.63,65 Dynamic changes in tumor blood flow, including treatment-initiated decreases in the blood flow, have been observed for several photosensitizers including porfimer sodium, 5-aminolevulinic acid (5-ALA; Levulan), motexafin lutetium (Lutrin), verteporfin (Visudyne), palladium bacteriopherophorbide (Tookad), and 2-(1-hexyloxyethyl)-2-devinyl pyropheophorbide-a (HPPH; Photochlor).63,66–72 In fact, PDT-triggered reductions in tumor blood flow during light delivery are not only therapy limiting, but also directly correlate with outcome measures. Yu et al.68 showed that the duration (long) and the slope (shallow) of the Photofrin-PDT–induced decrease in tumor blood flow correlated with the time-to-tumor regrowth (prolonged) in an animal. Similarly, Standish et al.73 and Pham et al.74 reported PDT-induced decreases in tumor blood flow and %StO2 (tissue hemoglobin oxygen saturation), respectively, correlated with necrosis development. These findings show the value in the development and application of noninvasive approaches to measure tumor blood flow during clinical applications of PDT.69,75,76 Thus, although PDT-induced ischemia can limit the effectiveness of PDT, in the setting of sufficient light and drug doses, the persistent ischemia that is induced can provide incremental cytotoxicity that can improve the effectiveness.47,77
Experimental strategies directed at modulating the vascular response to PDT are also consistent with the importance of its contribution to the PDT effect. For example, inhibitors of nitric oxide have been effective in increasing tumor and vascular damage to porfimer sodium or ALA-PDT in a protocol-dependent manner.78–80 Also, the vascular disrupting agent, vadimezan (5,6-dimethylxanthenone-4-acetic acid; DMXAA) has been successful in improving PDT responses when administered in such a way as to decrease tumor perfusion after illumination.70,81 It is even possible to deliver PDT in two fractions so that a vascular-damaging protocol follows one in which oxidative damage to tumor cells is the major cytotoxic mechanism.19 This approach has been studied in preclinical models using verteporfin as photosensitizer by first employing a longer interval between drug administration and light delivery to allow drug accumulation in the tumor cells, leading to direct light-induced cytotoxicity, followed by a second round of drug administration and light delivery that utilizes a short drug-light interval, which causes more extensive vascular damage due to drug localization in the blood vessels at the time of light delivery.19 Similar observations have been reported with the photosensitizer MV6401.26
Tumor Stress Response to Photodynamic Therapy
The oxidative stress initiated with PDT can induce the expression of genes that can mediate various modes of cell death as well as survival signals. Using c-DNA microarrays, the cellular effects of hypericin-mediated PDT was studied on the expression of a panel of genes involved in apoptosis, metabolism, and proliferation, among other cellular processes.82 Twenty-five genes were significantly up-regulated by PDT, including dual specificity phosphatase-1 (DUSP1), which can induce apoptosis; the stress response proto-oncogenes, FOSB and JUN, whose protein products dimerize to contribute to the transcription factor AP-1, another effector (both positive and negative) of apoptotic response; and MYC, which codes for the pro-apoptotic transcription factor c-myc. Among the genes down-regulated by PDT were THBS1, whose protein product thrombospondin-1 is an effector of cell interaction with the extracellular matrix, angiogenesis, apoptosis, and cell migration; ADAM10, which codes for a family of cell surface proteins with roles in epithelial cell–cell adhesion, migration, and proliferation; and several genes in the integrin family that mediate cell-to-cell and cell-to-matrix attachments, thereby facilitating signal transduction along pathways controlling apoptosis and metastasis, among other functions.82
In other studies, PDT-induced apoptosis was associated with activation of p38 in the mitogen-activated protein kinase (MAPK) family,83–85 which along with its other family members (including ERK and JNK) regulate cell proliferation, differentiation, and survival. Furthermore, expression of pro-apoptotic and anti-apoptotic proteins of the Bcl-2 family can also be modulated by PDT in a context-dependent matter, which depends on factors such as cell type and subcellular localization of photosensitizer.83,86,87 Similarly, PDT-induced expression and phosphorylation of survivin, capable of inhibiting apoptosis through inhibiting caspase-9, has also been an active area of investigation to therapeutically improve PDT cytotoxicity.88 Signal transduction in PDT-induced apoptosis, autophagy, and necrosis is an area of much and expanding interest, and for the interested reader, several comprehensive reviews43,89,90 are available on the current state of knowledge on this topic.
Immunologic Response to Photodynamic Therapy
The altered microenvironment generated by PDT can also serve to stimulate the host immune responses. Both the activation of an innate (nonspecific) and an adaptive antigen-specific immune response may be seen following PDT. These findings have raised considerable hopes that a localized treatment with PDT may lead to broader and possibly systemic oncologic benefits. For example, PDT-induced damage is associated with the local influx of neutrophils and other cell types, such as macrophages, natural killer cells, and dendritic cells, which contribute to local damage and inflammation while also initiating a broader systemic immune response.91 Both preclinical and clinical studies show this to be accompanied by the release of immune-modulating factors, such as interleukin (IL) 1-β, tumor necrosis factor (TNF)-α, IL-6, IL-10 and granulocyte colony-stimulating factor (G-CSF).92,93–94
The resulting activation of the innate immune response plays a major role in tumor control after PDT, and neutrophils are key to this process. In vitro studies demonstrate neutrophil adhesion to an extracellular matrix exposed by PDT-mediated endothelial cell damage.95 In vivo studies find neutrophils to attach to PDT-treated blood vessels,96 while various approaches toward depleting neutrophil influx into the PDT-treated tissues have been to the detriment of therapeutic outcome.97,98 In fact, a systemic neutrophilia is known to accompany PDT of various tumor types, sites, and photosensitization protocols.99–101 PDT can also activate the complement system with opsonization and fixation of the complement C3 protein to tumor cells, which also promotes a strong neutrophilia. This serves to not only target cells for destruction by the innate immune system but can induce the release of pro-inflammatory mediators further contributing to the migration of neutrophils.102
In addition to the induction of an innate immune response, PDT can also stimulate an adaptive cell-mediated immunity. In fact, these types of immune responses are intricately connected processes. It has been proposed that a critical aspect of PDT-induced adaptive immunity is the generation of a high antigen load with tumor cell death that is presented for adaptive immunity. Tumor cell death is further promoted by the strong neutrophilia through its release of lysosomal enzymes, including myeloperoxidase and the generation of further ROS. This strong neutrophilia also appears to be a critical factor for the development of PDT-induced adaptive immunity,103 as neutrophils degranulate and release a family of mediators referred to as alarmins. Alarmins are a critical link between the innate inflammatory response and adaptive immunity as they are capable of recruiting and activating the maturation of antigen-presenting dendritic cells.104 With a strong innate immune response, this antigen presentation can be more effectively recognized, inducing an adaptive cell-mediated immunologic response and memory.105
Dendritic cells (DCs) typically exist in the immature state within the tissue microenvironment actively surveying and capturing antigens then migrating and presenting these to T cells in adjacent draining lymph nodes. For dendritic cells to mature and affect adaptive immunity, the expression of costimulatory molecules that are involved in antigen-presentation to T cells is important. Several aspects of this innate response, including the strong neutrophilia103 and the release of damage-associated molecular patterns (DAMPs), consist of various markers of normal tissue injury. Among these, the extracellular release of heat shock protein-70 (HSP-70) and its association with tumor antigens by PDT-treated cells appears to be particularly effective in being recognized by DCs through surface receptors leading to their activation and maturation.106
HSPs, in particular HSP-70, have been a DAMP of particular interest in PDT for some time.107 HSPs are molecular chaperones crucial for proper protein folding. HSPs can facilitate cell survival during intracellular functioning, but become immunostimulatory when extracellular or membrane bound.108 In studies of PDT, HSP-70 is rapidly exposed on the surface of tumor cells treated in vitro,109 and its antibody-based blockage served to inhibit maturation of dendritic cells.110 Moreover, surface or extracellular expression of HSP-70 after PDT has been shown to correlate with curative outcome in temoporfin-treated murine tumors.111 Research in these and other aspects of PDT-generated antitumor immunity has spawned interest in the use of PDT to develop cancer vaccines, the history and progress of which have been recently reviewed.112
Vascular Response to Photodynamic Therapy
Despite the therapeutic benefits to be gained from PDT-created vascular damage and inflammation, it comes at a cost. In a post-PDT tumor microenvironment characterized by inflammatory infiltrates, cytokine overexpression, eicosanoid production, and hypoxia, there is a strong pro-angiogenic stimulus to support the growth of new tumor blood vessels.113 Such angiogenesis can counteract the intended effects of treatment by providing a means for delivery of oxygen and nutrients to tumor cells that escaped direct (oxidative) or secondary (vascular or immune-mediated) damage by PDT. Vascular endothelial growth factor (VEGF) is one of the most common angiogenic molecules whose expression is stimulated by PDT, and its increase has been measured following PDT with a variety of photosensitizers and tumor models.114–116 Moreover, studies of human tumors grown as murine xenografts find PDT to induce modest increases in host-derived (mouse) VEGF in addition to the increases in human VEGF that originate from the treated tumor.117,118 The molecular mechanisms of PDT-initiated increases in VEGF can include increases in hypoxia-inducible factor (HIF)-1α, a transcription factor that promotes the activation of many hypoxia-responsive genes such as VEGF,119,120 as well as activation of the p38 MAPK pathway.121 In the case of the latter, an inhibitor of p38 MAPK significantly attenuated the PDT-induced increase in VEGF.121
Cyclo-oxygenase (COX) 2 is another pro-angiogenic molecule that is up-regulated by PDT under a variety of treatment conditions.122,123–124 The COX-2 enzyme serves to catalyze the production of prostaglandin (PG) H2, a substrate for multiple eicosanoid mediators (including additional prostaglandins and thromboxane) known to contribute to PDT-created ischemia.125,126 The pro-angiogenic activity of COX-2 can be mediated through the enzyme’s role in production of PGE2, which in turn can promote increases in VEGF.113 Moreover, PDT-stimulated pro-inflammatory cytokines such as IL-1β and TNF-α may also stimulate angiogenesis through a COX-2 dependent pathway. This is supported by findings that decrease in PGE2 after COX-2 inhibition is accompanied by a reduction in protein levels of IL-1β and TNF-α.127
Biologic Strategies to Improve Photodynamic Therapy Cytotoxicity
With a growing understanding of the biological and molecular mechanisms that underlie PDT-derived cytotoxicity has come the development of alternative, more effective approaches toward PDT delivery. These include the development of new targeted PS that exploit specific signatures or functions in diseased tissue in order to deliver, or even to activate, the PS.128 These are commonly referred to as PDT molecular beacons, where the PS and a singlet oxygen-quenching or -scavenging molecule are both coupled to a linker that can interact with a cancer-specific target.129 In this way, the PS photoactivity is silenced due to the proximity of the singlet oxygen-quenching molecule. The PS is only capable of being photoactivated when the linker interacts with the cancer-specific target, which results in physical separation of the singlet oxygen-scavenging molecule from the PS. Examples of novel targeted linker constructs include an antisense oligonucleotide complementary to a target messenger RNA that is conformationally restricted until the oligonucleotide interacts with its target.
Modulation of light delivery has also proven successful in mitigating the microenvironment limitations imposed by PDT. For example, lowering the fluence rate of light delivery can conserve tumor oxygenation during PDT, increasing direct tumor cell cytotoxicity as well as vascular and immune effects.56,58,97,130,131–132 Similarly, fractionation of the light with or without repeat administration of the PS before each light fraction has improved treatment response in various preclinical protocols46,133–134,135–138 and in early clinical studies.139 Without repeat PS administration, preclinical studies suggest that the light fractionation is improving oxygenation46 with more effective vascular injury138 and necrosis.134 The duration of the light that is first administered133 and the duration of time between each light fraction134 may be particularly important in improving the oxygen delivery between light fractions. When the PS has been readministered before the second light fraction, a short DLI has demonstrated improved tumor control in preclinical models through vascular targeting with various photosensitizers such as verteporfin,19 MV6401,26 and m-tetrahydroxyphenylchlorin (mTHPC).140
As described above, PDT causes cytotoxic oxidative stress and induces the expression of pleiotropic pro-survival molecules such as COX-2.84 Understanding the mechanisms leading to pro-survival molecule induction is relevant to the design of more effective treatments. PDT in combination with various targeted agents designed to reduce the effect of the tumor stress response has demonstrated improved tumor responses in various preclinical models. Antiangiogenic agents lead to decreases in VEGF expression after PDT,115,141–143 along with improvements in tumor response.114,115,117,119,141–144 Inhibition of HSP increases the curative potential of PDT through decreased expression of angiogenic and pro-survival proteins in the treated tumors,145 while disruption of HSP-90 function in vitro leads to increases in PDT-induced apoptosis.88 The COX-2 pathway has also been targeted in combination with PDT and can improve therapeutic outcome through inhibition of post-PDT angiogenesis, as well as, under some circumstances, through increases in direct PDT cytotoxicity.127,146–148
PRACTICE OF PHOTODYNAMIC THERAPY
Light Delivery and Dosimetry
The ability to achieve PS activation is dependent on effective administration of light not only with a wavelength that matches the spectral absorption of the PS, but also on depositing a sufficient amount of energy to the target tissue (total fluence). Wavelengths >800 nm are unable to deposit sufficient energy to activate a PS. Delivering sufficient fluence is influenced not only by the technique of its administration (i.e., surface vs. interstitial), but also by the ability of the light energy to penetrate sufficiently at depth to treat the intended target volume. As light photons interact with human tissues, photons scatter and are absorbed by endogenous chromophores such as hemoglobin, myoglobin, melanin, and cytochromes. Hemoglobin is especially important to consider. Hemoglobin has spectral absorption peaks <600 nm (i.e., hemoglobin absorbs all colors except red), and its ability to absorb light energy is affected by its oxygenation status. Oxygenated hemoglobin is less likely to absorb between 600 and 800 nm compared to deoxygenated hemoglobin. Thus, most activating light that has been used for PDT has typically been between 600 and 800 nm, depending on the spectral absorption characteristics of the PS.
The rate at which the light energy is delivered (fluence rate) is also an important treatment factor that can affect the efficacy of PDT. It primarily affects the tissue microenvironment such as the vascular flow and oxygenation. In general, it is important to recognize that high fluence rates can rapidly consume and reduce the local oxygen levels such that it limits the efficacy of the remaining light fluence.149 Although the prescribed fluence rate is typically based on the power output of the light source, surface and internal scattering of light photons may result in areas of higher fluence rates. Strategies to reduce at least the surface scatter effect or to modify the prescribed power output of the light source should be considered (see below).
At present, the prescription of PDT typically used clinically remains limited to rudimentary power output calculations for surface illumination:
where the prescription dose is given in terms of the energy per unit area incident on the surface.
Various light delivery devices have been developed to perform PDT treatment. Most of them are fiber based. These include linear source, endotracheal-tube–modified point source, collimated light source, and flat-cut fiber (Fig. 27.2). The flat-cut and linear sources are suitable for inserting into the tissue for interstitial PDT application for the treatment of bulky tumors. The collimated light source is suitable for superficial treatment.
It is therefore helpful to recognize that various illumination techniques may be employed depending on the geometry of the target lesion that is to be treated. The most common clinical situation requires surface illumination, where several technical approaches may be considered. Where the geometry of the target lesion is flat or may be modified to be a near flat surface, the use of a light fiber with a diffusing lens at the tip of the fiber (i.e., microlens) offers the ability to achieve homogeneous light distribution across the surface target. Inhomogeneities due to different distances between points on the surface of the target and the light source can result in different fluence rates and the total light dose (fluence) that is effectively delivered. Unlike ionizing radiation, the surface scattering of photons due to surface concavities or reflective surfaces (i.e., any adjacent metal surfaces) can further increase the risk of high surface dose inhomogeneities. In a similar manner, surface convexities may create regions of shadowing that can create regions of low fluence and fluence rate. Areas of high light fluence (and its fluence rate) may also contribute to an increased risk of normal tissue complications.
When the target lesion is cylindrical, a cylindrical diffusing fiber with the light dose prescribed along its length has commonly been used. However, it is important to note that where the target surface is not rigid (i.e., esophagus), mucosal folds that are not in apposition to the light fiber may become underdosed. To reduce this risk, the cylindrical diffusing fiber can be placed within a balloon diffuser that can be expanded to increase its surface apposition with the mucosal surface. Similar considerations can be applied to spherical surface targets such as the mucosa of the bladder.
The use of a balloon diffuser may be helpful for certain complex three-dimensional surfaces, such as the lateral oral tongue and its adjacent floor of mouth where the surface to be treated can be molded in apposition to the balloon diffuser. In such situations, it is important to verify that the mucosal surface is in direct contact with the balloon’s surface before the light is delivered. Other strategies for such complex three-dimensional surfaces may include dividing the target volume into separate targets and individually treating each area with a microlens (patching technique). With this approach, it is important to bear in mind that areas of potential overlap, when illuminated, may increase the risk of normal tissue complications. Other surface illumination techniques under development include a light blanket that attempts to mold the light source to such complex three-dimensional surfaces.
Interstitial light fibers can also be placed when volume illumination is required. As with interstitial brachytherapy techniques, the geometry of the light fibers can significantly affect the overall distribution and amount of light that is delivered. Thus, the use of rigid templates guiding the insertion of the trocar needles (Fig. 27.3) can be very helpful in ensuring accurate interfiber spacing. These templates may be used to facilitate the advancement of rigid trocar needles whose track can then be replaced with light fibers. Alternatively, traditional low-dose rate after-loading plastic catheters may be used instead to allow the use of the needle track for both light detection fibers or treatment fibers where prescription is based on light dosimetry. The placement of the catheters can also facilitate several quality assurance measures. This can include verifying the geometry of the implant, allowing additional catheters to be placed or removed to optimize the geometry. It may also include verification or modification of the location of the light fiber in its catheter relative to the tumor volume.
Whether the optical properties of the tissue being treated are different by staging the placement of the implant and its illumination is not clear. However, where significant tissue trauma occurs with the placement of the implant, staging the illumination may offer some potential advantages especially where significant tissue bleeding with the introduction of the trocar needles has occurred. Other theoretical advantages may also include improved oxygenation of the implanted tissue, both improving the photosensitization process and reducing hemoglobin absorption of the activating light energy.
Although the optimal interstitial PDT prescription parameters remain to be defined, it is heartening to see successful interstitial light implants having been reported in patients. For such results to become generalizable, the development of a robust and easy-to-use dosimetry system will be needed that will facilitate characterizing the impact of different prescription factors (i.e., intercatheter distance, fluence rate) on normal tissue complications and oncologic results.
FIGURE 27.2. Various fiber-based light sources. These include a linear source (A), an endotracheal-tube modified point source (B), collimated light fiber (C), and a flat cut fiber (D).
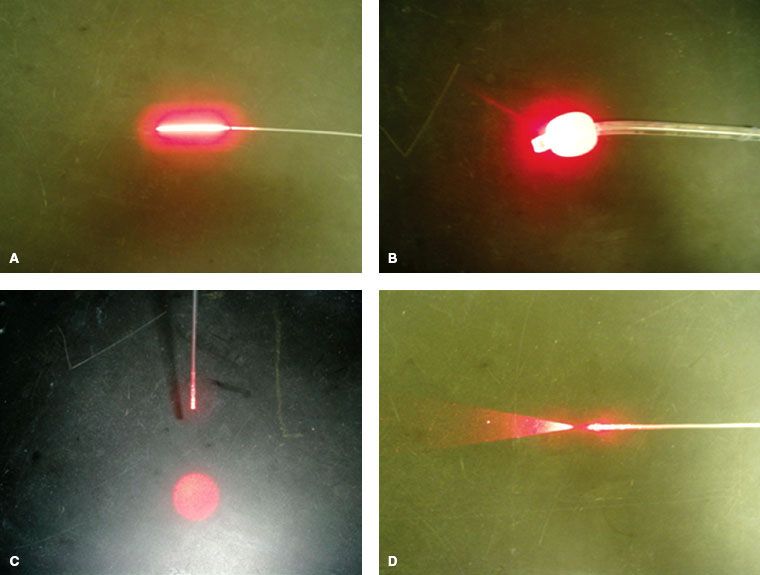
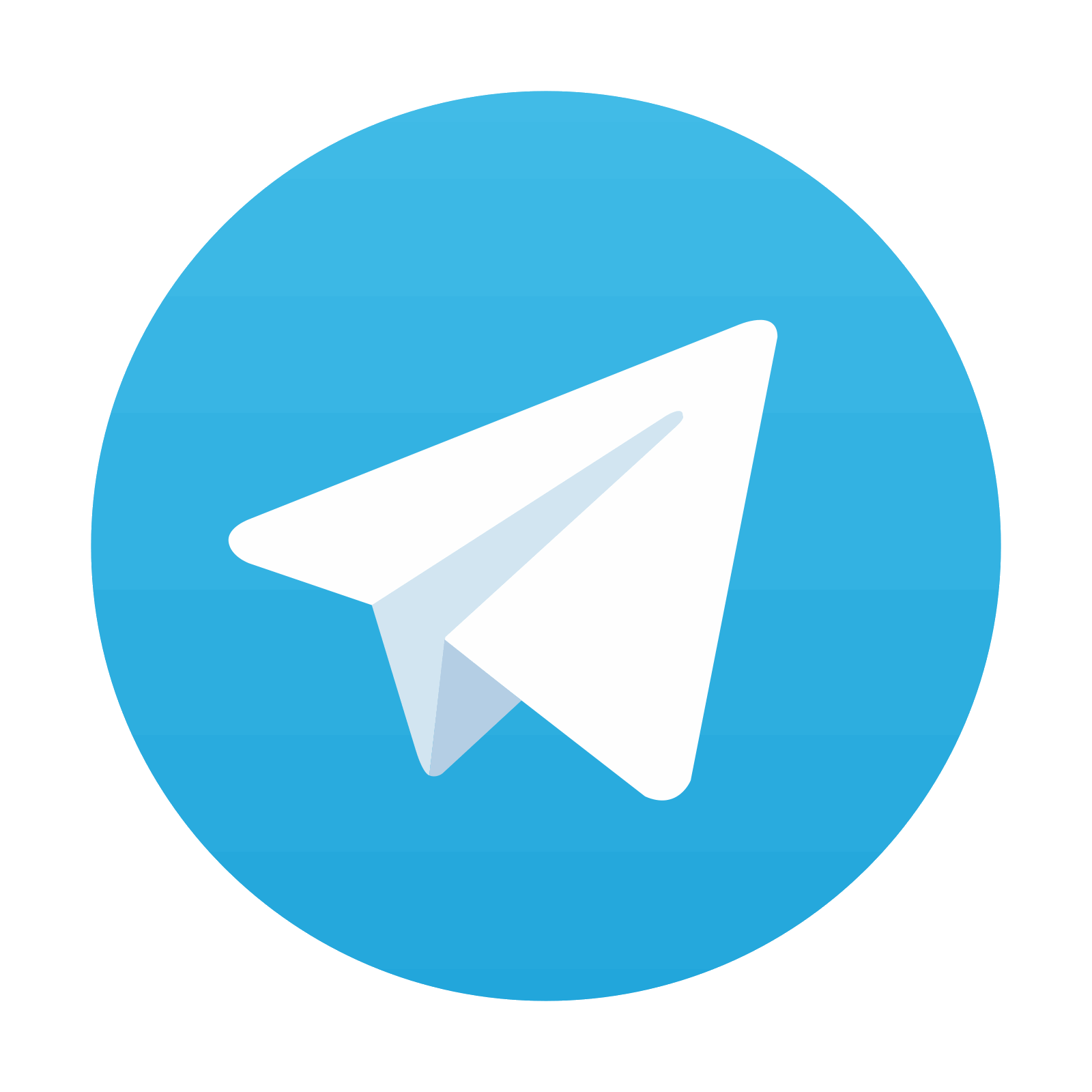