6.1
Developmental origin of osteoblasts
6.1.1
Control of osteoblast function
6.1.1.1
Signaling pathways that control bone formation
Osteoblasts originate from mesenchymal stromal cells (MSCs) that represent the primary cells that support the formation, remodeling, and repair of bone tissue. In lineage tracing experiments, MSCs are marked by α-smooth muscle actin (α-sma/ACTA2) promoter activity that represents a key subpopulation that eventually gives rise to osteoblasts by the intricate molecular interplay of multiple signaling pathways that control osteogenic lineage commitment and progression . The pathways that control these processes are activated by a range of extracellular protein ligands (e.g., growth factors and morphogens), including WNT , bone morphogenetic protein (BMP)/transforming growth factor β (TGFβ) , parathyroid hormone (PTH) , fibroblast growth factor (FGF) , epidermal growth factor (EGF) , insulin-like growth factor (IGF) , and NOTCH . These signaling pathways promote the activities of a number of transcription factors that modulate the stage-specific expression of bone-related collagenous (e.g., COL1A1 and COL1A2) and noncollagenous proteins (e.g., osteocalcin/BGLAP, osteopontin/SPP1, bone sialoprotein/IBSP, alkaline phosphatase/ALPL).
6.1.1.2
Transcriptional mechanisms that regulate osteogenic lineage commitment and osteoblast differentiation
Osteogenic signaling pathways ultimately activate principal transcription factors that stimulate and/or sustain the osteoblast or osteocyte phenotype. A large number of transcription factors have been identified that are considered critical for induction of osteoblast-specific gene expression. This extensive list includes (but is not limited to) the following proteins: Runt-related transcription factor 2 (RUNX2), the Kruppel-like factors (KLFs) SP7/Osterix and TIEG1/KLF10, the homeodomain proteins DLX5, DLX3, MSX2, SATB2, HOXA10, CDP/CUX1 and TGIF1, the basic leucine-zipper (bZIP) proteins FOS/JUN (AP1; FOS, FRA1, FRA2, JUN, JUNB JUND), ATF4, CEBPB and CEBPD, the helix-loop-helix (HLH) proteins TWIST1 and HEY1, as well as the nuclear hormone receptors: vitamin D receptor (VDR), retinoid acid receptor alpha (RXRA), estrogen receptor (ERRA), glucocorticoid receptor (NR3C1). The literature on bone-related transcription factors is vast, and for other perspectives on this subject, readers are encouraged to read additional reviews on this topic .
Differentiation- and proliferation-related functions of the bone master regulator factor, Runx2. Runx2 is the most well-known osteogenic transcription factor, because genetic loss of Runx2 results in a boneless mouse with only a cartilaginous skeleton . The osteoblast-specific transcriptional role of Runx2 was initially inferred from its identification as nuclear matrix protein 2 that was found to bind to multiple sites in the osteocalcin promoter, as well as subsequently as an osteoblast-specific nuclear factor (OBSC) and osteoblast-specific factor (OSF2) . Prior to the now well-established finding that Runx2 is an osteogenic lineage commitment factor, Runx2 was initially discovered and rediscovered several times in the cancer field as (1) a key protein required for polyoma tumor virus replication (i.e., polyoma enhancer-binding protein 2 alpha 1), (2) retroviral core-motif binding factor alpha that is associated with retroviral induction of murine leukemia, and (3) the gene activated by retroviral integration at the T-cell lymphoma integration locus (Til-1). This profusion of names for the same factor was an impediment to understanding the early literature on this protein, but the nomenclature was standardized by the adoption of universal gene symbols for the three RUNX proteins present in mammalian genomes .
The involvement of Runx2 in supporting virally induced cancers is consistent with cell growth regulatory roles of Runx2 in osteoblasts and osteosarcomas that complement its lineage-commitment functions. In normal osteoblasts, constitutive overexpression of Runx2 suppresses osteoblast growth, presumably by preventing the reduction of Runx2 levels that occurs prior to S phase and by establishing a nonproliferative state (e.g., senescence or quiescence). For example, cell growth suppression by Runx2 is evidenced by the observation that primary calvarial cells from Runx2 null mice proliferate faster than wild-type osteoblasts, and reexpression of Runx2 into these cells suppresses this accelerated cell growth. Gene expression profiling has been used to define Runx2 targets required for its growth and differentiation potential. Among the many Runx2 targets that support its cell growth regulatory potential are G-protein coupled receptors and downstream signaling components, the cytochrome protein Cyp11a1, components of the FGF signaling machinery, and the RNA helicase Ddx5. The ability of Runx2 to control proliferation is cell type specific and depends in part on the status of p53 and/or Myc. Runx2 inhibits proliferation of calvarial osteoprogenitors and committed osteoblasts, but in a diverse array of cancer cells (e.g., osteosarcoma, T-cell lymphoma, breast, and prostate cancer cells), it may either promote tumor growth or metastasis.
From a molecular perspective, Runx2 can both activate or repress its target genes and as such represent a bifunctional transcription factor. Runx2 represents the nuclear end-point effector of many if not all osteogenic pathways. It mediated the integration of multiple signaling pathways by interacting with several dozen cofactors and protein modifying enzymes. Cbfb is the most well-known cofactor of Runx2 and increases the DNA-binding activity and protein stability of Runx2 . Runx2 activates genes in response to the TGFβ/BMP2 serine kinase signaling pathway by directly interacting with SMAD proteins, or in response to Src/Yes tyrosine kinase signaling by interacting with Yap/Taz proteins. Repression of Runx2 is supported by binding to GROUCHO/TLE proteins that interact with a highly conserved C-terminal VWRPY pentapeptide in Runx2. Furthermore, RUNX2 interacts with a number of enzymes, including the lysine deacetylase HDAC, the prolyl isomerase Pin1, the ubiquitin-conjugating protein SMURF1, and many others. The reversible acetylation and phosphorylation of Runx2 may represent key drivers of the overall protein stability and activity of the protein.
To accommodate the growth regulatory activity of Runx2, the expression of this protein is modulated by transcriptional and posttranscriptional mechanisms during the cell cycle at both mRNA and protein levels. Specifically, Runx2 protein is expressed at the highest levels in early to mid-G1 and downregulated prior to initiation of S phase . This downregulation of Runx2 protein is controlled in part by phosphorylation during G1 by Cyclin D1 (CCND1)/CDK4 complexes that promote ubiquitination of Runx2 and subsequent proteasomal degradation . Although Runx2 protein levels are minimized prior to S phase, the protein is maintained at a low sentry level in S phase, G2 phase, and mitosis (M phase). This minimal basal level of Runx2 is phosphorylated during the G2 and M phases of cell cycle by cyclin B (CCNB1 or CCNB2)/Cdk1 complexes that may modulate the regulatory activity of Runx2 during mitosis. Importantly, Runx2 protein is retained on mitotic chromosomes and as such bookmarks osteoblast-related genes. The latter represents a noncanonical epigenetic mechanism (architectural epigenetics) that may establish a developmental memory of the committed phenotype when osteoblast progenitors undergo mitotic division . This mechanism may reestablish the osteoblast-specific phenotype by the rapid activation and repression of Runx2 target genes in early G1, independent of external osteogenic regulatory cues (e.g., WNT, BMP, or FGF signaling).
Remarkably, even though Runx2 mRNA and protein are both maintained at relatively low basal levels during S and G2 phases, the mRNAs of Runx2 are elevated again in late G2 just prior to mitosis, even though its protein levels are not upregulated until after mitosis in G1 . This preloading of daughter cells with mRNAs that are produced prior to mitosis ensures that cells do not have to activate the Runx2 gene by the intricate process of transcriptional activation but rather can simply begin rapidly producing Runx2 proteins by translating the existing pool of Runx2 mRNA. As such, the mitotic programming of osteoblasts with preexisting Runx2 mRNAs provides a mechanism for changing the reliance on transcriptional signaling pathways into a dependency on translational signaling pathways.
Translational control of Runx2 is mediated in part by microRNAs (miRNAs), and the long 3′UTR of Runx2 mRNA has seed sequences for many tens of miRNAs . There are at least a dozen miRNAs, which interact with this 3′UTR and have been shown to control Runx2 protein and mRNA expression in osteoblasts. Interestingly, the effects of Runx2 regulatory miRNAs are cell type specific, and some miRNAs are more active in osteoblastic cell lines, whereas others appear to work better in chondrocytic cell lines.
The expression of Runx2 is controlled by two promoters, P1 and P2, that generate two different mRNAs . The utilization of the two different promoters fuses two different starting exons to the main body of the Runx2 protein. The N-termini of Runx2 are therefore somewhat different: the N-terminal pentapeptide MRIPV is derived from an exon transcribed from the P2 promoter, while a ~20 amino acid N-terminus starting with MASNS is derived from the P1 promoter. Functional differences have not been convincingly demonstrated, and it is likely that these proteins are in fact functionally indistinguishable. It is conceivable that the P1 and P2 protein isoforms may differ in protein stability, because the two isoforms may conform to the “N-end rule” that predicts protein stability based on the penultimate amino acid. The P1 and P2 have remarkable differences in base pair distribution. The P2 promoter is highly GC rich and contains many CpG doublets that permit methylation of the 5 position of cytosine (epigenetic 5mC DNA mark). In contrast, the P1 promoter is more AT rich and devoid of CpG doublets. The P1 promoter responds to osteogenic signaling pathways such as WNT and BMP signaling. Hence, the bone-specific P1 promoter is a developmentally activated regulatory region, while the P2 promoter may be regulated primarily by the formation of 5mC marks. Bone-specific transcription of Runx2 is mediated by a novel enhancer region in the P1 promoter that is stimulated by Dlx5/6, Mef2, Tcf7, Ctnnb1, Sox5/6, Smad1, and Sp7 .
Runx2 expression is also controlled by posttranscriptional regulatory mechanisms. For example, the 5′UTR of the Runx2 P1 mRNA is relatively short and AT rich, with limited potential for secondary 3D structures (e.g., hairpins). The Runx2 P2 mRNA is very long, GC/rich, and heavily burdened by extensive predicted secondary structures. The model that emerges from these observations is that the P2 mRNA is regulated by reversible methylation. As such, the P2 mRNA is rather constitutively transcribed but inefficiently translated, unless the cell was to activate a mechanism to remove hairpins (e.g., via putative Runx2-related RNA helicases) or uses an internal ribosome entry mechanism. In contrast, the P2 promoter is induced in a more flexible developmental stage-specific manner, but its translation is maximally efficient due to the absence of secondary structures that would impede ribosomal progression.
Sp7/Osterix as the primary target osteogenic target of Runx2 . Sp7/Osterix is one of the most definitive early markers of the osteoblast lineage. Similar to Runx2, genetic knockout (KO) of Sp7 creates a boneless mouse because it acts immediately downstream of Runx2. Sp7 is a member of the SP1 family of GC-box-binding proteins (e.g., SP1, SP3, and SP7) that are part of a larger superfamily that includes other KLFs (e.g., KLF4 and KLF10). The regulatory potential of this class of proteins to affect stem cell fate and differentiation potential is perhaps most easily illustrated with KLF4, which is one of the key genes required for reprogramming lineage-restricted somatic cells (e.g., skin fibroblasts) into pluripotent stem cells. Analogous to the discovery of Runx2, the closely related SP1 protein, which is a housekeeping protein that is ubiquitously expressed in many cell types, was initially identified as a stimulatory factor required for the activation of early gene expression of the DNA tumor virus SV40 . In contrast to Runx2 that is broadly expressed in many mesenchymal cell types except mature resting chondrocytes, SP1 is ubiquitously expressed in many cell types, whereas SP7/Osterix is primarily expressed in committed osteoblasts and hypertrophic chondrocytes. The osteoblast-related expression of SP7 renders its promoter very valuable as a transcriptional driver of the Cre recombinase in conditional KO models. SP7 is a unique BMP-inducible member of the SP/KLF superfamily, and as such is together with Runx2 considered a master regulator of BMP-mediated osteoblast differentiation.
TIEG1/KLF10 is an estrogen-dependent modulator of the bone phenotype . TGFβ-inducible early growth response protein 1 (TIEG1) is an estrogen-responsive KLF that stimulates Runx2 , as well as the TGFβ- and BMP2-dependent transcription of the Osterix/SP7 gene in osteoblasts. Similar to RUNX2, this protein is capable of suppressing the growth of osteoblasts . More recently, TIEG1 was found to regulate canonical Wnt signaling via effects on β-catenin stabilization and nuclear localization . Notwithstanding these important regulatory effects of TIEG1 on WNT signaling and BMP2 signaling, the KLF10/TIEG1 KO mouse has a rather mild, gender-specific, low bone mass phenotype that (as expected from its estrogen dependence) is only penetrant in females , compared to the dramatic effects of RUNX2 and Osterix/SP7 KOs.
Regulation of the bone-specific gene expression program by the homeodomain proteins DLX5, DLX3, MSX2, HOXA10, CDP/CUX1, SATB2, and TGIF1 . Multiple homeodomain transcription factors have effects on craniofacial or skeletal development that were initially identified as regulators of the bone-specific osteocalcin/BGLAP gene. Of these, DLX5 is quite interesting because it is a BMP2-responsive homeodomain protein that controls both RUNX2 and the osteocalcin/BGLAP promoter . In addition, genetic KO of DLX5 affects the development of the craniofacial bones . Similar findings were made with the closely related protein DLX3, which also controls the osteocalcin/BGLAP gene, and genetic mutation of DLX3 alters bone mass . Dissecting regulatory mechanisms controlled by DLX proteins is complex, and the closely related members DLX4 and DLX6 also have roles in craniofacial development and skeletogenesis . The homeodomain-binding sites for DLX proteins are located at a conserved motif in the proximal promoter of the osteocalcin/BGLAP promoter that is also recognized by the homeodomain proteins MSX2 and CDP/CUX1 , and their functions appear to be restricted to immature osteoblasts. MSX2 null mutations are linked to craniofacial abnormalities , whereas CUX1 null mice did not have overt skeletal abnormalities . Additional roles in osteoblastogenesis are evident for the canonical homeodomain protein HOXA10 that controls both skeletal patterning and osteoblastogenesis . The nuclear matrix-associated SATB2 homeodomain protein, which is similar to CUX1, is a multifunctional protein with conserved cut repeats as auxiliary DN-binding domains. The homeodomain protein TG-interacting factor 1 (Tgif1) was more recently described as a transcriptional antagonist of PTH signaling that is required for bone remodeling . The large number of homeodomain proteins that control skeletal development and osteoblast differentiation reflects the complexities of skeletal patterning that are required for proper formation of the many bony elements in the mammalian skeleton.
Control of osteoblastogenesis by bZIP transcription factors, HLH proteins, and nuclear hormone receptors . AP1/ATF-related transcription factors, which are hetero- and homodimers composed of FOS, JUN, and ATF, as well as the CEBP class of factors, were each initially identified as regulatory proteins that bind to the osteocalcin promoter. The AP1-binding site in the osteocalcin promoter overlaps with a vitamin D-responsive element and may functionally interfere with VDR binding. Prominent members of the AP1 family in osteoblasts are FRA2, JUNB, and JUND. The proximal region of the osteocalcin promoter interacts with ATF4, as well as CEBPB and CEBPD. Twist1 and the NOTCH signaling responsive Hey1 protein each of which is known to bind to RUNX2 or the RUNX2 gene promoter provide a direct mechanistic explanation for their activities in bone formation. Control of bone-specific gene expression is also mediated by VDR, which enhances tissue-specific basal levels of osteoblast-related genes together with its heteromeric partner RXRA. ERRA controls osteoblastogenesis by facilitating expression of KLF10, while NR3C1 supports dexamethasone-dependent induction of osteoblastogenesis.
6.1.1.3
Epigenetic regulation of bone formation and osteoblastogenesis
Transcription factors control MSCs and osteoblasts function by recruiting epigenetic regulators that support the remodeling of nucleosomal organization. Induction of the osteoblast-specific gene expression during bone formation is mediated by the conversion of dense facultative heterochromatin into chromatin with a more open configuration. The opening of chromatin involves removal or conversion of 5-methyl cytosine DNA methylation and/or posttranslational modifications (PTMs) of histone proteins, including acetylation, methylation, and/or monoubiquitination of lysines (K). Other known modifications are serine (S) phosphorylation (e.g., histone 3; H3S10) and arginine (R) methylation. Modifications of 5-cytosine in DNA are generated by DNA methyltransferases, while DNA hydroxylases (i.e., the “ten eleven translocation” enzymes; Tet1, Tet2, and Tet3) modify 5mC residues into 5hmC modifications. 5mC levels in promoters typically repress genes, while the presence of 5hmC typically signifies open active chromatin. As a general rule for histone PTMs, stimulation of transcription involves H3 and H4 acetylation, as well as H3K4 and H3K79 methylation, while methylation of H3K9, H3K27, and H4K20 results in gene repression. The proteins that generate, interpret, and remove (or edit) these histone PTMs are informally referred to as, respectively, histone writers, readers, and erasers of the histone code. The biochemical enzymes include histone methyltransferases at lysine or arginine residues (KMT/PRMTs) and the corresponding lysine or arginine demethylases (KDM/PRDMs). Acetylation marks are controlled by acetyltransferases (KAT/HATs) and deacetylases (HDAC/SIRTs), as well as histone serine kinases, phosphatases. Some histone residues are modified by more than one mark and biochemically altered by about a dozen different enzymes. In total, histone PTMs are controlled by as many as 300 different epigenetic enzymes.
Epigenetic regulators of DNA . Tet proteins control musculoskeletal development by reactivating genes silenced by 5mC modifications into 5hmC modifications in the regulatory regions of genes. At least one Tet protein is necessary for live animals with a normal skeletal body plan. Each of the three Tet genes (Tet1, Tet2, and/or Tet3) has both distinct and redundant biological roles during skeletogenesis based on the phenotypes of mice with null mutations in one or more Tet genes . At present, there are no definitive data on the specific functions of Tet proteins in bone formation, but RNA interference assays for the three Tet proteins have revealed that each of these proteins contributes to osteoblast differentiation.
Epigenetic regulators of histone proteins . Histone acetylation correlates with active transcription, and this PTM is countered by HDACs. Null mutations for three HDACs (i.e., Hdac1, Hdac3, and Hdac7) have been shown to cause skeletal abnormalities and osteopenia . Acetylation of lysines in histones H3 and H4 is recognized by bromodomain (BRD) proteins that act as histone readers. Recent results indicate that BRD4 controls skeletal homeostasis by modulating both osteoclastogenesis and osteoblastogenesis . BRD proteins may control bone-specific transcription by binding to osteoblast-specific promoters and enhancers, as well as interactions with CDK9, a kinase that stimulates transcription by engaging RNA polymerase. Drugs that inhibit BRD4 activity may promote accumulation of bone mass in adult mice, suggesting that BRD4 inhibition could be considered for treatments to counter bone loss (e.g., in osteoporosis). Histone methylation by polycomb repressive complex 2 (PRC2) and its catalytic subunit “enhancer of zeste homolog 2” (EZH2) EZH1/2 has been shown to be important for normal skeletogenesis and bone formation. PRC2 mediates H3K27 trimethylation (H3K27me3) and this histone mark is important for altering chromatin into a highly dense and transcriptionally silent configuration . However, osteoblastogenesis requires the activation of genes that are in such suppressed and transcriptionally inactive heterochromatin. Therefore inhibition of this enzyme complex promotes bone formation and osteoblast differentiation. Mice with conditional loss-of-function mutations in Ezh2 exhibit a range of phenotypes depending on the stage at which the gene is inactivated. Loss of Ezh2 in MSCs decreases limb growth and accelerates osteogenesis in the calvarial sutures, as well as having other effects that are also evident in human patients with Weaver syndrome . Remarkably, Ezh2 appears to be mostly dispensable in cartilage presumably due to compensation by the closely related Ezh1 protein. Molecular studies revealed that removal of Ezh2 increases transcription of Bmp2-responsive genes (e.g., Osx/Sp7) and many classical bone proteins (e.g., Ibsp and Bglap) . Taken together, bone formation is controlled by multiple distinct epigenetic regulators. Modulating the biochemical activities of these proteins may alter chromatin structure in a manner that promotes bone-specific gene expression and osteoblast functions to support new bone formation.
Epigenetic regulation by noncoding RNAs . Osteoblast-specific gene expression is controlled at the posttranscriptional level by small noncoding RNAs referred to as miRNAs. These regulatory RNAs control translation and/or stability of mRNAs encoding both osteoblast- and osteoclast-related proteins to control both bone formation and homeostasis . A large number of regulatory interactions between mRNA–miRNAs in bone have been described . Pathological changes in the expression and function of miRNAs have been linked to age related, degenerative bone diseases, including osteoporosis . miRNAs primarily suppress gene expression and one main function of miRNAs is to provide feedback control. Negative feedback emerges by activation of miRNAs that suppress expression of proteins required to support stimulatory signals . This negative function permits molecular resetting of osteoblast-related pathways at the completion of physiological processes. In addition, miRNAs can provoke positive stimulation when they target suppressors of cell signaling pathways and/or gene regulation (stimulation via “double-negative” regulation) . MiRNAs are currently considered both as relatively stable biomarkers and potential RNA therapeutics for the treatment of disorders of low bone mass. Beyond small noncoding RNAs, there is accruing evidence for the importance of long noncoding RNAs (lncRNAs) in bone formation and osteoblast differentiation. Recent studies identified hundreds of differentially expressed lncRNAs during osteogenic differentiation of MSCs. It is therefore likely that lncRNAs may emerge as another class of critical regulators of osteogenesis and bone homeostasis .
6.1.2
Morphology
6.1.2.1
Osteoblasts
Based on morphological and histological studies, osteoblastic cells are categorized in a presumed linear sequence progressing from osteoprogenitor cells to preosteoblasts that mature to osteoblasts and then to lining cells or osteocytes ( Fig. 6.1 ). There is a gradient of differentiation that can be observed morphologically either in the periosteum or in the marrow as the osteoprogenitor cell reaches the bone surface and the osteoblast phenotype becomes fully expressed. Preosteoblasts are usually observed as one or two layers of cells behind the osteoblasts near bone-forming surfaces; that is, they are usually present where active mature osteoblasts are laying down bone matrix. They appear elongated, fibroblastic, or spindle shaped with an oval or elongated nucleus and with notable glycogen content. Preosteoblasts may express a few phenotypic markers of the osteoblast, for example, alkaline phosphatase (ALPL) activity, but less than mature osteoblasts. The preosteoblast, however, has not yet acquired many of the differentiated characteristics of mature osteoblasts; for example, there is no evidence of a well-developed rough endoplasmic reticulum.

When the preosteoblast ceases to proliferate, a key signaling event occurs for the development of the mature osteoblast from the spindle-shaped osteoprogenitor. The osteoblast expresses all of the differentiated functions required to synthesize bone. Osteoblasts are defined in vivo by their appearance along the bone surface as large cuboidal cells actively producing matrix ( Fig. 6.1 ), which is not yet calcified ( osteoid tissue ). Several structural features characterize the osteoblast, including its size and cuboidal morphology, a round nucleus at the base of the cell (opposite to the bone surface), a strongly basophilic cytoplasm, and a prominent Golgi complex located between the nucleus and the apex of the cell . At the ultrastructural level , one observes an extremely well-developed rough endoplasmic reticulum with dilated cisternae and dense granular content, and a large circular Golgi complex consisting of multiple Golgi stacks. These are typical characteristics of a secretory cell.
Mature osteoblasts that are derived from proliferating osteoprogenitors can be observed in clusters at the bone surface ( Fig. 6.1 ). These cells synthesize the bone extracellular matrix (ECM), designated osteoid ( Fig. 6.1 ). In metabolic bone disorders leading to decreased calcium or phosphate deposition in bone, as in vitamin D deficiency, wide osteoid seams are evident. Mineralization leads to the final stage of osteoblast differentiation. When the bone-forming osteoblast becomes encased in its own mineralized matrix, they have become osteocytes.
Four different entities of the osteoblast cell lineage are thus recognized in vivo. They are the committed progenitors (preosteoblasts), mature osteoblasts, osteocytes, and the bone-lining cell. Yet, knowledge on the mechanisms determining whether bone-forming osteoblasts become osteocytes, bone lining cells or undergoing apoptosis, is limited.
6.1.2.2
Osteocytes
As the active matrix-forming osteoblasts become embedded in the mineralized matrix, the cells differentiate further into osteocytes that comprise 90%–95% of all bone cells . The osteocyte and its functions are extensively described elsewhere in this volume (Bonewald chapter). Most osteocytes are passively entrapped but some osteocytes also actively migrate into the matrix by activating collagen cleaving matrix metalloproteinases (MMPs) . The osteocyte is considered to be the mechanosensor of bone tissue reflecting its primary function to maintain bone as a viable tissue supporting physiological needs and structural requirements. Labeling studies suggest that the transition from an osteoblast to an osteocyte takes approximately 3–5 days . Mechanisms that induce the osteocyte morphology to a smaller cell body with numerous cytoplasmic extensions are not understood , but transitional stages are recognized in vivo . The osteocyte is considered the most mature or terminally differentiated cell of the osteoblast lineage, not capable of cell division in vivo. Osteocytes are embedded in bone matrix occupying spaces ( lacunae ) in the interior of bone ( Fig. 6.1 ) and are connected to adjacent cells by long cytoplasmic projections radiating from the cell body. These dendritic processes are enriched in microfilaments and lie within channels ( canaliculi ) through the mineralized matrix and form gap junctions with processes of neighboring cells and cells lining the bone surface, that is, osteoblasts and osteoclasts. Mice lacking metalloproteinase MT1-MMP have significantly reduced number and length of these dendritic processes but osteocyte density and viability seem unaffected . In vitro, the markers of the osteocyte associated with dendritic extensions have identified cell lines with preosteocyte and mature osteocyte properties . The lacunae and the canaliculi together constitute the so-called lacuna–canalicular network (LCN). Studies on osteocytes and the morphology of the LCN have been hampered by the inaccessibility of these cells as they are buried in the bone matrix. Nijweide et al. were the first to isolate osteocytes from avian bone by using a specific antibody . More recently, isolation of primary osteocytes from murine long bones in skeletally mature and aged animals was established . In isolated cultures, mature osteocytes retain their cellular projections . Through different gap junction proteins called connexins, described earlier, osteoblasts and osteocytes are coupled metabolically and electrically. Rapid fluxes of calcium across these junctions facilitate the transmission of information between osteoblasts on the bone surface and osteocytes within the structure of bone . The osteocytes and surface-lining cells form a continuum, or syncytium, by connection of their cytoplasmic projections through gap junctions that facilitate the exchange of both mechanical and metabolic signals for responsiveness to physiologic demands on the skeleton. More recently, the use of genetic models and sophisticated imaging modalities have allowed for a more detailed analysis of the LCN and the vascular canal network that has yielded new insights in osteocyte morphology, density, and effects of aging hereon .
6.1.2.3
Bone lining cells
On a quiescent bone surface the osteoblast flattens to a lining cell being part of an endosteum ( Fig. 6.1 ). Bone lining cells are in direct communication with osteocytes within the mineralized matrix through cellular processes that lie within the canaliculi. The lining cells are considered to provide a selective barrier between bone and other extracellular fluid compartments and contribute to mineral homeostasis by regulating the fluxes of calcium and phosphate in and out of bone fluids . The bone lining cells have been shown to play a role in the bone remodeling process by cleaning the Howship lacunae and initiating bone formation . The bone lining cells may also play an important role as part of the stem cell niche and in tumor metastasis to the bone . Although it is believed that osteoblasts derive predominantly from bone marrow–resident MSCs, recent lineage tracing studies have shown that lining cells constitute a major source of osteoblasts during adulthood. Following ablation of osteoblasts in mice, bone lining cells are recruited to differentiate into new osteoblasts, expressing markers that are shared with MSCs . Glucocorticoid treatment in this mouse model reduced bone mass, pointing to a potential role for bone lining cells in glucocorticoid-induced osteoporosis . In contrast, a different osteoblast lineage tracing study showed that intermittent PTH treatment activated periosteal bone lining cells into cuboidal osteoblasts that synthesize osteoblast marker genes . These studies emphasize that bone lining cells are not simple bystanders on quiescent bone surfaces but are actively involved in bone metabolism by transforming into active osteoblasts.
6.1.3
Extracellular matrix
The primary functional activity of the surface osteoblast is production of an ECM with competency for mineralization. The bone ECM is a very dynamic collagenous tissue that harbors and actively modulates the availability and signaling of several growth factors, such as bone morphogenetic proteins. As a consequence, the ECM modulates MSC, osteoblast, and osteocyte behavior, including cell adhesion, proliferation, and commitment . This is due to the enrichment of proteoglycans and enzymes such as ALPL and MMPs in the ECM. In addition, a wide range of noncollagenous proteins, such as osteonectin, osteocalcin, osteopontin, and matrix gla proteins, regulates cell adhesion/matrix attachment and collagen fiber mineralization . In fact, the proteome of human bone and that of ECM generated by human osteoblasts consists of many different collagenous and noncollagenous ECM proteins .
6.1.3.1
Collagenous proteins
The major matrix component synthesized by osteoblasts is collagen type I that compromises nearly 90% of bone protein and provides the essential substrate for mineral deposition. The osteoblast synthesizes and vectorially secretes most of the bone ECM protein; others are accumulated. Fetal bone is enriched in type III collagen, whereas type I collagen accounts for 80%–90% of the osteoid in the adult with minor amounts of collagen type V. Collagen forms a fibrillar network stabilized by unique cross-links to maintain structural integrity of the tissue upon mineralization . The fibrils organize with precise spacing that can accommodate deposited mineral. Discrete sites in the collagenous matrix serve as initial sites of mineral deposition in the hole regions between end-to-end collagen fibrils , accommodating small specialized bone proteins that interact with collagen and function as nucleators of hydroxyapatite. Collagen determines the structural organization of trabecular and cortical bone (woven, lamellar, haversian architecture) and supports the flexibility of mineralized tissues. Obviously, conditions that alter the collagen composition or orientation, for example, hypoxia, affects ECM assembly and mineralization . Collagen and the highly specialized noncollagenous proteins, which are either synthesized by the osteoblast or derive from other tissues and accumulate in bone bound to collagen and/or mineral, contribute to mineralization of the osteoid, bone structure, and bone tissue metabolic functions.
6.1.3.2
Noncollagenous proteins
The noncollagenous groups of proteins represent components of the bone ECM function in mediating cell signaling from the ECM, cell adhesion/matrix attachment, protein–protein interactions by binding to collagen to regulate fibrillogenesis, as well as controlling mineral deposition through nucleation and inhibitor activities. Immunostaining identified a broad range of ECM proteins, including growth factors, such as TGFβ and IGF in the mineralized bone matrix while most often the osteoid was negative, suggesting that these are only produced and secreted into the matrix just prior to mineralization . Noncollagenous proteins have been classified by their functional protein domains and PTMs. These include proteoglycans, RGD-containing proteins, glycoproteins, γ-carboxyglutamic acid (gla)-containing proteins as well as the small integrin-binding ligand N-linked glycoprotein (SIBLING) proteins and phosphoproteins. Osteonectin, osteocalcin, bone sialoprotein, osteopontin, and dentin matrix protein-1 (DMP1) were long considered the most abundant noncollagenous proteins in the ECM. However, a recent mass spectrometry proteomic analysis of human bone tissue delivered a human bone proteome library of over 1200 proteins . Most classical bone matrix proteins mentioned in the literature were detected but, interestingly, were not among the highly abundant ones. Gene ontology analyses identified high-abundance groups of proteins with a functional link to mineralization and mineral metabolism such as transporters, pyrophosphate (PPi) activity, and Ca 2+ -dependent phospholipid-binding proteins. Whether these proteins have a functional role in the ECM and/or bone metabolism remains to be elucidated.
Ablation of the genes encoding some of the more abundant and bone-restricted noncollagenous proteins [osteocalcin , osteopontin , biglycan (BGN) ] have resulted in only subtle changes in the bone matrix and mineral phase of bone that were not anticipated from in vitro studies and the calcium- and phosphate-binding properties. However, the phenotypes are revealing their functions and structural components for bone tissue integrity. The glycosylaminoglycan chains of decorin (DCN) and BGN facilitate their strong association with hydroxyapatite . Deletions of the DCN and BGN genes in mice disturb collagen fibril organization. Bgn null mice have progressively diminished bone mass with age, while Dcn -deficient mice have normal bone mass. However, the BGN/DCN double KO mice have severe osteopenia . The importance of BGN in bone was shown by forced overexpression, which accelerated osteoblast differentiation in vitro and in vivo transplanted cells resulted in large areas of lamellar bone . BGN potentiates BMP2 signaling in murine osteoblasts, which is limited by the glycosaminoglycan residues in BGN. BMP2 signaling and bone formation are potentiated more by deglycanated BGN than glycanated BGN .
Osteonectin (secreted protein acidic rich and cysteine rich; SPARC) is a glycoprotein bound to collagen and expressed by osteoblasts that are actively depositing matrix. Mice lacking Sparc exhibit osteoporosis and decreased osteoblast number and bone formation consistent with alterations in collagen structure, mineral composition, and crystal morphology . Sparc -deficient mice revealed increased osteoclastogenesis following PTH treatment compared to WT mice, but the mechanism behind the protective effect of SPARC is currently unknown . SPARC, together with a.o. osteopontin/SPP1, bone sialoprotein/IBSP, tenascin C, and the thrombospondins 1 and 2, is an example of the matricellular proteins. These are part of the ECM and are linked to regulation of cell function and gene transcription . Osteonectin, osteopontin, and bone sialoprotein were found to be coupled to transglutaminases . Transglutaminases have extracellular cross-linking activity and, thereby, promote the osteoblast adhesive properties of matricellular proteins .
A membrane protein that could function in coupling the ECM to the cytoplasm and nucleus and be a component of the cellular tensegrity model is neuronal membrane glycoprotein (GPM6B). Knockdown of GPM6B affected the cytoskeleton and inhibited osteoblast differentiation and induction of mineralization Support for a role of GPM6B in intracellular signaling in osteoblasts is derived from the observation that silencing GPM6B significantly downregulated expression of PDLIM7 (PDZ and LIM domain 7 also known as LMP1, LIM mineralization protein 1). PDLIM7 has been shown to be involved in BMP6-stimulated osteoblast differentiation and mineralization . Antisense block of PDLIM7 expression prevented osteoblast differentiation. GPM6B may function as a membrane-localized switch in osteoblasts that integrate the ECM-derived signals into an intracellular signal and alters osteoblast function and, eventually, bone formation.
Several of the SIBLING noncollagenous proteins synthesized by the osteoblast and osteocyte are upregulated during osteoblast differentiation and participate in ECM mineralization . IBSP, a phosphorylated glycoprotein with a hydrophilic domain that binds to collagen, is expressed nearly exclusively in bone . IBSP binds to hydroxyapatite through polyglutamic acid regions required for its functional activity as a nucleator of hydroxyapatite . Ibsp null mice have higher trabecular bone mass with a low bone turnover phenotype, increased cortical bone porosity, and impaired response to unloading . Matrix extracellular phosphoglycoprotein (MEPE) functions as a regulator of inorganic phosphate (Pi) concentration, and the null mouse exhibits increased bone mass and density , indicating an inhibitory role of MEPE in osteoblast activity and mineralization . Disruption of MEPE expression in osteoblasts leads to increased bone formation and bone mass . Osteopontin/SPP1 is a protein with a wide distribution with many functions, including an inhibitor of bone mineralization and ectopic calcification . This protein tends to be enriched on surfaces undergoing bone turnover and provides an interacting protein module for adherence and activity of the bone-resorbing osteoclast . Thus facilitating osteoclastic bone resorption is an important SPP1 function . Spp1 null mice have a subtle phenotype , but technologies for resolution of crystal size and maturity in null mutant mouse models reveal defects in bone mineral and quality. Increased mineral content and maturity (i.e., perfection) was found throughout all anatomical regions of the Spp1 -deficient mouse bone, consistent with the osteopontin function in bone resorption/turnover . In contrast, to the Ibsp null mice, the Spp1 null mice do not lose bone upon hind limb unloading pointing to an impaired mechanosensitivity . Mice lacking both Bsp and Spp1 display a complex bone phenotype revealing additional roles for both SIBLING proteins and their interaction in bone metabolism .
DMP1 is another SIBLING protein highly expressed in osteocytes. It is an acidic (glutamic and aspartic acid rich) phosphoprotein that functions in stimulating osteoblast differentiation, as well as responding to mechanical loading . A recent intriguing observation was that DMP1 is also detected in the nucleus of osteoblasts suggesting a yet to be proven role in intracellular signaling . The phenotype of mice lacking Dmp1 resembles the human condition autosomal recessive hypophosphatemic rickets (ARHR) and is characterized by elevated levels of the osteocyte-produced phosphaturic hormone FGF23 along with reduced trabecular and cortical bone mass . The rachitic bone phenotype revealed a marked increase in unmineralized osteoid matrix as a consequence of the low circulating phosphate , but also phosphate responsiveness is affected . Interestingly, the multiphosphorylated proteins, characterized by stretches of serines, which include SPP1, IBSP, DMP1, and MEPE, all map to the q arm of chromosome 4 .
Osteocalcin/BGLAP accumulates in relation to mineral deposition. It is a vitamin K-dependent protein necessary for synthesis of its three calcium binding γ-carboxyglutamate residues (Gla). Gla residues promote BGLAP binding to hydroxyapatite. This property, as well as its upregulation by 1,25(OH) 2 D 3 , suggests a dynamic role in calcium deposition and mobilization. Inactivation of the Bglap gene did not result in a major skeletal phenotype during development and growth, but after 4 months, a higher bone mass was observed compared to WT without a change in osteoblast number . The crystal properties of Bglap -deficient mice differ from WT . While the osteocalcin precise function remains obscure, over 5000 papers have documented BGLAP as a valued serum marker of bone turnover, and a marker of the mature osteoblasts whose expression correlates with matrix mineralization. Observations from mouse genetic studies revealed a role of uncarboxylated Bglap in regulating pancreatic insulin secretion and target cell insulin sensitivity as well as in male fertility, in memory functions driven by maternal osteocalcin pools and in physical exercise . These observations put forward regulation of carboxylation as an important osteoblast activity with broader implications than only bone metabolism. Whether this is true in humans remains to be proven as the data from human studies are inconclusive. Uncarboxylated BGLAP levels can be influenced by confounding factors such as vitamin K status that have to be taken into account. Therefore well-controlled clinical studies have to be performed to take this intriguing observation in mice to the human .
From the genetic studies related to bone matrix proteins thus far, it appears that inactivation of genes representing the abundant noncollagenous proteins leads to subtle defects in the skeleton, suggesting that no one protein is a major determinant of mineralization and bone. The implication of the genetic studies is that the noncollagenous proteins may have redundant or coordinated functions, and that each of their specialized functions is contributing to the properties of the mineral phase. This concept is supported by the observation that inhibition of mineralization by the growth factor activin A is paralleled not by a change in a specific noncollagenous matrix protein but by a change in the gene expression profile of multiple noncollagenous proteins suggesting that the combination and the ratio of the noncollagenous proteins is vital for controlled ECM maturation and mineralization .
6.1.3.3
Matrix mineralization
Mechanisms for facilitating hydroxyapatite deposition specifically in bone matrix are multifactorial. While substrate requirements for mineralization of bone cannot be underestimated, initiation of hydroxyapatite formation must also be considered in the context of (1) the organization of ECM components, (2) enzymes required to support an environment for nucleation, and (3) mineral homeostasis for appropriate mineral composition. Early inductive events for nucleation involve (1) removal of inhibitors of mineralization (ATP, PPi, citrate, and proteins) by enzyme activities; (2) mechanisms for raising local calcium and phosphate ion concentrations; and (3) propagation of hydroxyapatite crystals from initial deposits, which occurs through epitaxy of initial crystallites mediated by the matrix (reviewed by Ref. ).
Matrix vesicles are specific organelles produced by osteoblasts and shed at the apical surface into the ECM . Matrix vesicles contain enzymes [e.g., alkaline phosphatase, ectonucleotide pyrophosphatase/phosphodiesterase 1 (ENPP1), PHOSPHO1, and MMPs], transport proteins (e.g., annexins), integrins, lipids, and ECM proteins . Studies indicated that matrix vesicles may be carriers of BMPs, vascular endothelial growth factor (VEGF) and noncollagenous matrix proteins . They also harbor genetic material (DNA, mRNA and miRNAs, etc.) and, being recently coined extracellular vesicles (EVs), have been implicated in paracrine communication or beyond . However, the purity and mineralization functionality of the vesicle preparation in this study as in other proteomic analyses of osteoblastic vesicles remain to be established as osteoblasts may secrete a broad range of vesicles (e.g., exosomes) other than matrix vesicles .
The removal of inhibitors involves two enzymes present in matrix vesicles that have been identified as central regulators of the mineralization inhibitor PPi: (1) ENPP1 that produces PPi from ATP and the nucleoside triphosphates, (2) ALPL that hydrolyzes PPi to Pi, and (3) PHOSPHO1 that hydrolyzes phosphoethanolamine and phosphocholine in the initial steps of matrix mineralization . In addition, a transmembrane protein ANKH (associated with ankyolosis) that transports intracellular PPi functions as a calcification inhibitor by increasing extracellular PPi .
Alkaline phosphatase activity is still considered critical to the initiation of mineralization, a concept supported by characterization of the genetic defect in hypophosphatasia . Generating the Alpl null mouse, Waymire et al. demonstrated that the mechanism of impaired mineralization of cartilage and bone in this mouse represents the defect of infantile hypophosphatasia . The ability of ALPL to cleave PPi, thereby removing the inhibitor, is an essential ALPL function . As a consequence, Pi is generated providing a local environment for nucleation and growth of the mineral phase as proven by in vitro studies of Alpl −/− osteoblasts that cannot initiate mineralization . Studies devoted to the role of PHOSPHO1 in Phospho1 -deficient mice have shown that it nonredundantly facilitates the initial step of mineralization by transporting Pi into the matrix vesicles to form hydroxyapatite, followed by the extravesicular mineralization process, that is dependent on by ALPL .
Consistent with deficiencies in ALPL that inhibit mineralization due to a rise in PPi levels, inactivating mutations in enzymes that produce PPi, the family of ectonucleotide pyrophosphatase/phosphodiesterases, exemplified by ENPP1, result in hypermineralization defects . Inactivation of the Ankh gene, which transports PPi, also led to a hypermineralization phenotype . An elegant series of studies of the genetic crosses of the Alpl −/− , Enpp1 −/− , and the Ank −/− mouse provided several lines of in vivo evidence that PPi is an inhibitor of mineralization and that mineralization occurs in bone as a result of the ability of ALPL to cleave PPi .
The abovementioned discussed SIBLING proteins regulate ECM mineralization. The Dmp1 KO mouse exhibits a hypomineralization phenotype in teeth and bone . The nonphosphorylated protein is a hydroxyapatite nucleator in vitro but exhibits inhibitory properties when phosphorylated. However, phosphorylated DMP1 peptides, which are isolated from bone and teeth, behave as nucleators . These findings support mechanisms for controlling hydroxyapatite formation, not only by protein phosphorylation but also through protein cleavage at specific aspartic acid sites. Through molecular approaches, it has become evident that multiple functional groups in noncollagenous proteins have nucleating and inhibitory activities. For example, by generating a chimeric protein that included the collagen-binding domain of DCN and the apatite-nucleating domain of BSP, the deposition of large needle-shaped crystals on collagen was far greater than with either of the control proteins . The inhibitory activity on mineralization of MEPE was localized to the C-terminal aspartic acid–rich motif (ASARM) , but a peptide fragment of MEPE containing the integrin-binding RGD and the glycosaminoglycan attachment sequence serine-glycine-aspartic acid-serine (SGDS) supported increased bone formation in in vitro and in vivo models (reviewed in Ref. ). In vitro, human osteoblast studies demonstrated that sclerostin (SOST) inhibits mineralization and increases MEPE expression. The inhibition of mineralization is antagonized by neutralizing antibodies against MEPE-ASARM implying that SOST inhibits mineralization via a MEPE-ASARM-dependent process .
Modifications in calcium and phosphate homeostatic mechanisms must be considered for an understanding of the mineralization pathologies that are associated with metabolic bone disease. Maintaining serum calcium levels through calciotrophic hormone axes [PTH, calcitonin, and 1,25(OH) 2 D 3 ] impacts on the bone reservoir. Bone will mineralize in a normal physiologic manner when serum calcium is maintained through adequate dietary absorption. Vitamin D deficiency or metabolic bone diseases associated with dysfunctional enzymes or receptors for the hormone 1,25(OH) 2 D 3 will lead to osteomalacia in adults and rickets in children and impaired bone formation in the mouse . Transgenic mice expressing two- or threefold higher levels of the VDR expressed in osteoblasts resulted in bone with a high calcium content compared to wild type with decreased bone resorption and increased homogeneity of the mineral deposits and collagen maturity . These findings are consistent with the importance of vitamin D for bone structural integrity and the anabolic effects of 1,25(OH) 2 D 3 on bone and are leading the way to better therapeutic approaches by ligand-specific modulation of the VDR/RXR receptor . In the case of intestinal malabsorption of calcium, maintaining normocalcemia prevails at the cost of vitamin D–induced inhibition of bone mineralization . Whether this observation in mice translates to the human situation remains to be established as discrepancies between human/rat and murine bone with respect to vitamin D have been reported. For example, vitamin D stimulates BGLAP expression in human and rat osteoblasts, while it inhibits Bglap expression in murine osteoblasts .
Pi is essential, in combination with calcium, for mineralization in bone and formation of the hydroxyapatite crystals. The majority of homeostatic regulation of Pi occurs through actions of renal Pi handling by PTH and its regulation of the 25-hydroxyvitamin D1 α-hydroxylase enzyme that increases levels of the active hormone, 1,25(OH) 2 D 3 . In addition, Pi regulatory proteins, called phosphatonins, have been identified through rare genetic disorders in humans, including the X-linked autosomal-dominant hypophosphatemic rickets (ADHRs) (XLH) and the ARHR and ADHR (reviewed in Ref. ). The hyp mouse representing the syndrome of XLH genetic defect was found to result from an inactivating mutation in an endopeptidase called PHEX, proposed to be functionally linked to a phosphatonin. Transgenic expression of PHEX in osteoblasts improved the defective bone mineralization in the hyp mouse but does not fully rescue the metabolic phenotype . The genetic basis of ADHR was identified to be a mutation in FGF23 that appears to have phosphatonin properties in that increased secretion will induce phosphaturia and hypophosphatemia . Mouse models of Fgf23 defined a key role in Pi metabolism with demonstration of an osteomalacia phenotype, implicating FGF23 in bone mineralization, or an indirect effect through the hypophosphatemia and high vitamin D levels . Progressive insights have led to a few proteins able to modulate FGF23 function, such as FAM20C, GalNT3, and Furin , but many questions remain. For example, if and how these proteins may be controlled by Pi, what the role of cations such as iron and potassium is in phosphate homeostasis, and how extracellular Pi is perceived? Intriguingly, a phosphate sensor, equivalent to the calcium-sensing receptor in bone, has yet to be found . In addition to a role for FGF23 in mineralization through phosphate homeostasis, a link may exist between FGF23 and the SIBLINGs. In tumor-inducing osteomalacias, FGF23 is expressed at abnormally high levels, as are two other proteins with apparent phosphaturic action: MEPE and frizzled-related protein 4 .
6.2
Interactive nature of osteoblasts
6.2.1
Cell–matrix interaction
MSC phenotypes and lineage allocation can be influenced by changing matrix elasticity . Soft matrices induce a more neurogenic phenotype, whereas rigid matrices that mimic bone surface induce an osteogenic phenotype. Commitment of a stem cell to a phenotype is regulated by cell shape and cytoskeletal changes that involve Rho GTPase activity. A dominant-negative RhoA promotes a round shape leading to adipocyte differentiation, while a constitutively active RhoA induced the osteogenic phenotype independent of cell shape . Physical forces on the MSC appear to be a significant component for osteoblast allocation as microgravity inhibits osteoblast colony formation of human MSCs and increases adipocytes . Furthermore, modifications of surface structures can specifically activate ECM gene expression, expression of cell adhesion molecules, and the secretion of growth factors . The ECM modulates the cellular characteristics of MSCs, osteoblasts, and osteocytes, including cell adhesion, proliferation, and commitment . Our current understanding of the importance of material stiffness, cell adhesion, and surface interactions in MSCs and osteoblasts may permit rational strategies for tissue-engineering applications to modify mesenchymal lineage commitment and osteogenic lineage progression in skeletal tissue-engineering applications.
The molecular pathways by which internal and external forces control osteoblast-related gene expression are currently being elucidated. Biomechanical stimuli alter the architectural properties of the actin cytoskeleton that are transduced to the nucleoskeleton via linker of nucleus and cytoskeleton (LINC) protein connections that modify nuclear shape and nuclear import of gene regulator proteins . Recent data support the exciting novel concept that actin polymerization and branching also occurs in the nucleus and performs a key function in the activation of bone- versus fat-related genes . Microscopy imaging and biochemical experimentation established that the nuclear actin is controlled by an “actin toolbox” of formins and actin-related proteins that dynamically control linear versus branched polymerization of actin, as well as control the structural functions of lamin B1 protein (LMNB) . In addition, modifications in nuclear actin organization have direct effects on epigenetic regulators (e.g., EZH2) that control chromatin structure and the osteogenic gene expression program by restriction transcription factor access . Thus biomechanical forces and nucleoskeletal control of gene expression are key components of molecular regulatory models for self-renewal and mesenchymal lineage allocation.
6.2.2
Paracrine actions
6.2.2.1
Osteoblast–osteoclast interaction
Biological functions of osteoblast lineage cells go beyond their role in bone growth (stromal osteoprogenitors), matrix production (osteoblasts), and structural integrity of bone (osteocytes). Importantly, all these cells respond to endocrine factors as PTH/PTHrP (parathyroid hormone-related protein) and 1,25(OH) 2 D 3 are released to meet physiologic needs for osteoclastic resorption of bone. The bone-forming cell populations then produce cytokines and coupling factors that are essential for the sequel of events mediating the growth and differentiation of osteoclasts. The mechanisms coupling osteoblast and osteoclast activities for regulated bone turnover are well documented due to an explosion of information in the past decade . Induction of bone resorption and turnover is initiated through the osteoblast, mediated by two key pathways: (1) indirect mechanisms by which calciotrophic hormones stimulate stromal cells and osteoblasts to secrete macrophage colony-stimulating factor (CSF) that promotes growth of hematopoietic precursors and activate osteoclastogenesis through binding to CSF 1 receptor (CSF1R) ; and (2) the RANK–RANKL {(receptor activator of nuclear factor kappa-light-chain-enhancer of activated B cells (NF-κB) and ligand), also known as TRANCE [tumor necrosis factor (TNF)α–related activated induced cytokine]} system that involves direct interactions between RANKL on osteoblast lineage cells and its receptor (RANK) on preosteoclasts to activate intracellular signaling cascades for osteoclast differentiation . A wide range of mouse models has established the pivotal role for RANKL and RANK in the way osteoblasts instruct osteoclasts (reviewed in Ref. ). In more recent work, leucine-rich repeat-containing G-protein coupled receptor 4 on osteoclasts has been proposed as an alternative receptor for RANKL . Other costimulatory factors, as the immunoreceptor tyrosine-based activation motif (ITAM) adaptor proteins, cooperate with RANKL to activate osteoclast differentiation. Mice lacking two ITAM adaptor proteins (DAP12 and Fc receptor gamma chain) are severely osteopetrotic . Together, both the RANK/RANKL and MCSF/CSF1R regulatory pathways are required for coupling stromal/osteoblastic cells to the formation of osteoclasts, which in turn are controlled by cytokine and hormonal mediators of bone resorption to govern bone turnover.
Following bone modeling during infancy and puberty, the resorption and formation of bone at a single site is designated the bone remodeling unit. A key negative regulator of osteoclast differentiation, also mediated by cross talk from osteoblasts, is secreted osteoprotegerin (OPG), a secreted protein with strong homology to the TNF receptor family. This soluble inhibitor of the RANKL–RANK interacts to ensure that bone formation predominates when required. Several experimental approaches established OPG as a soluble factor competent to inhibit osteoclast differentiation by blocking the RANKL/RANK interaction . Expression of the OPG gene in osteoblast lineage cells is upregulated by calcium and downregulated by the glucocorticoid, dexamethasone . In addition to the RANK–RANKL–OPG system, the Toll-like receptor 9 on osteoclasts and osteoblasts mediates CpG oligodeoxynucleotide signaling for regulation of osteoclastogenesis . Also, in vitro and in vivo studies show P2Y nucleotide receptors mediate intercellular calcium signaling between osteoblasts and osteoclasts to regulate bone formation and bone resorption . Other examples include monocyte chemoattractant proteins, Wnt5a and Wnt16, semaphorin 3A, factor associated suicide ligand, and lysophosphatidic acid (reviewed in Ref. ).
In basal condition, expression of RANKL in osteoblasts is low and probably needs induction by hormones, growth factors, and mechanical loading. The actual source of RANKL is yet unclear as multiple cells in the bone and bone marrow, including osteocytes, bone marrow stromal cells, and lymphocytes, can express RANKL . Elegant genetic experiments showed that RANKL expression is higher in osteocytes than in osteoblasts and that specific deletion of RANKL expression in osteocytes but not T-lymphocytes led to impaired osteoclast formation and an osteopetrotic phenotype . Osteoblasts produce a range of cytokines, including interleukin-1 (IL-1), IL-6, and interferons (IFNs), that also can regulate osteoclast formation. A recent example is IL-33, which is produced by osteoblasts, and inhibits osteoclast formation while enhancing osteoblastic mineralization .
Newly identified factors, secreted from osteocytes and osteoclasts, appear to function in maintaining a balance between resorption and formation. The Eph/ephrin system has come forward as an important communication system within the bone environment between bone cells but also between bone cells and other cells in the bone marrow. For example, osteoclasts express ephrinB2, while osteoblasts express its receptor ephrinB4. This signaling from ephrinB2 suppresses osteoclast differentiation, while ephrinB4-initiated signaling enhances osteogenic differentiation, implying that the ephrin signal system appears to be essential for bone homeostasis . Other osteoclast-derived factors that modulate osteoblast function, include but are not limited to, BMP6, sphingosine-1-phosphate, the d2 isoform of the vacuolar proton ATPase (v-ATPase) V0 domain, and semaphorin 4D/Plexin B1 (reviewed in Refs. ). Also, calcitonin appears to play a crucial role in osteoclast to osteoblast communication as it inhibits bone resorption but at the same time controls bone formation by the inhibition of sphingosine-1-phosphate synthesis by osteoclasts . SOST is unique in its own way, as the osteocyte-produced bone mass inhibitor simultaneously activates osteoclasts and inhibits osteoblasts within bone .
Having been recognized as messengers between cell types, miRNAs have been implicated to communicate between osteoclasts and osteoblasts. For example, osteoclast-derived miR-214-3p was shown to modulate osteoblast function, and that this was mediated through its packaging into EVs .
6.2.2.2
Osteoblasts in the hematopoietic stem cell niche
While the interrelationship of bone tissue cells with the hematopoietic lineage cells in modulating bone resorption is well established, cross talk of osteoblasts with other systems is emerging ( Fig. 6.2 ). It is now well established that osteoblasts constitute a central regulatory part of the hematopoietic stem cell (HSC) niche . Osteoblasts retain HSCs at the endosteal surface through interaction of the chemokine SDF-1 (CXCL12) and its cognate C-X-C chemokine receptor type 4, allowing for their renewal . When HSCs are mobilized and proliferate, they move into the reticular and perivascular niches of the bone marrow microenvironment where stromal progenitor cell populations, such as nestin and leptin receptor positive MSCs and blood vessel-surrounding CXCL12-abundant reticular cells, bind HSCs to prepare them for exiting the bone marrow niche . In fact, there is a reverse staging interaction between osteoblast stages and HSC differentiation: the most differentiated osteoblast stage, being located on the endosteal surface, retains stemness of the HSC, whereas early osteogenic progenitors/MSCs circulating in the bone marrow instruct the proliferating HSCs (reviewed in Ref. ). When osteoprogenitors undergo genotoxic stress, the bone marrow microenvironment may undergo changes that lead to myelodysplasia and the emergence of acute myeloid leukemia. This was exemplified by knocking out the ribonuclease Dicer in these cells, leading to profound bone effects but also to severe myelodysplasia, events that did not take place in the case of Dicer -deficient mature osteoblasts . Intriguingly, in Shwachman–Diamond syndrome, mutations in the ribosome synthesizing SBDS gene lead to myelodysplastic syndrome (MDS). Mechanistically, faulty osteoblasts produce damage-associated S100A8 and S100A9 molecules, which in turn lead to mitochondrial dysfunction, oxidative stress, and DNA damage in HSCs, ultimately leading to MDS .
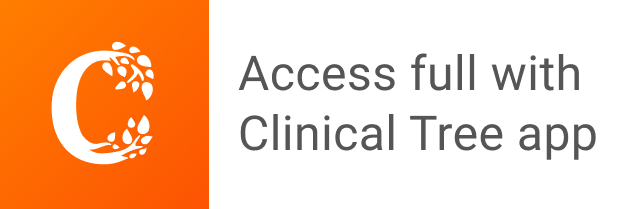